Introduction
Facial aging is complex and occurs at all tissue levels, from the underlying skeletal scaffolding, through the musculature, adipose tissue, and skin. Advances in aesthetic medicine now allow us to not only recognize specific changes in these tissue layers, but also address and in some cases correct the clinical signs of aging at its respective anatomic site.
The skin ages through two main processes: intrinsic and extrinsic aging. Intrinsic aging is the result of genetic background and chronology. It is an inevitable consequence of aging seen in all anatomic locations of skin regardless of exposure to ultraviolet (UV) light. Changes characteristic of intrinsic aging include decreased elasticity, decreased skin turgor, and loss of hair. On histology, the skin demonstrates flattened rete ridges and dermal atrophy . Extrinsic aging of the skin is the result of external factors such as UV exposure, alcohol use, tobacco use, and nutrition. These are largely controllable factors and therefore extrinsic aging is not necessarily inevitable. UV exposure is the primary driver of extrinsic aging, with some estimates attributing over 80% of facial skin aging to the sun . Extrinsically aged skin appears primarily in sun-exposed areas such as the face, neck, chest, and extensor arms, and is often referred to as “photoaging”. Clinically it appears as rhytids, dyschromia, lentigines, loss of elasticity, skin fragility, telangiectasias, and purpura. In more severe cases actinic keratoses and skin cancer begin to develop.
Aging of the skin, particularly that of photoaging, is readily apparent to both patient and practitioner and a common reason to visit a cosmetic physician. Because the pathologic process of photoaging is primarily limited to the epidermis and upper dermis, it is readily amenable to treatment with a wide variety of lasers and light sources. In 2014, over a half a million laser skin resurfacing procedures were performed in the United States, a 218% increase from 2000 . The remainder of this chapter will address the use of lasers to resurface the skin and treat signs of aging. The focus of this chapter is to review the principles and clinical applications of lasers in the treatment of aging skin. A full understanding of the physical properties of lasers used in medicine allows the practitioner to best adjust the settings to each patient and clinical application.
Laser Principles
The word laser is an acronym for L ight A mplification by S timulated E mission of R adiation. Lasers contain four key components: (1) an excitable medium; (2) an energy source; (3) mirrors forming an optical cavity; and (4) a delivery system. When energy is applied to the lasing medium, photons are emitted that stimulate further photon emission equal in energy and wavelength. This results in the emission of photons that are in phase both temporally and spatially; thus, the light emitted by a laser is one single wavelength, or monochromatic. This is a key attribute of lasers and why devices that emit a range of wavelengths, like intense pulsed light, are not lasers but should be referred to as light sources. Emitted light from lasers is completely in phase, meaning all peaks and troughs of the wave are in line. Thus, the light is termed coherent. This property allows the laser to be focused to a spot size as small as the wavelength itself. Additionally, the beam of light from a laser is collimated, meaning it travels in a parallel, nondivergent path unlike that of traditional light sources
Skin Optics and Selective Photothermolysis
When light from a laser strikes the skin there are four possible interactions: (1) reflection; (2) scattering; (3) transmission; and (4) absorption. Reflected light is redirected away from the skin and therefore no tissue effect occurs. However, the reflected light can cause ocular harm to those around. Reflection is least when light strikes perpendicular to the skin. Scattering of light occurs when the beam meets tissue and spreads in different directions. Scattering limits the effective depth of penetration of lasers. In general, larger spot sizes have less scatter, resulting in a greater depth of penetration. Transmission is the direct, unaltered penetration of light through tissue. The final light-skin interaction is absorption. This is where the energy of the photon is absorbed by the skin, leading to effect on the tissue. This is the desired effect when delivering a therapeutic treatment.
Absorption within the skin occurs when light strikes a chromophore. The main chromophores in the skin are hemoglobin, melanin, and water. When absorption occurs, a photon transmits its energy to the chromophore and generates heat. Thermal coagulation, if controlled and confined, can lead to selective destruction of a variety of targets in the skin.
In 1983, Anderson and Parish published a seminal paper on the theory of selective photothermolysis. They described how with proper laser parameter selection, one could achieve confined thermal damage of a target structure while leaving the surrounding tissue intact. To occur, the wavelength of light must be selectively absorbed by the target chromophore and be emitted for a duration less than or equal to the thermal relaxation time of the target. This allows for the confinement of heat. Thermal relaxation time of a molecule is directly related to the size and shape of the target. This theory has led to the development of numerous lasers with specific targets and clinical applications.
History of Laser Resurfacing
Treatment of photodamaged skin with laser resurfacing has been the gold standard for rejuvenation for over 30 years. Fully ablative resurfacing with continuous-wave carbon dioxide (CO 2 ) or erbium:yttrium-aluminum-garnet (Er:YAG) gained significant popularity in the early 1990s. The primary chromophore for these devices is water. There are several absorption peaks for tissue water, as demonstrated in Fig. 6.1 . Er:YAG emits light at 2940 nm, allowing for precise vaporization of tissue with minimal residual thermal damage. This is termed tissue ablation. CO 2 lasers emit light in the far infrared spectrum at 10,600 nm and are also absorbed well by water, resulting in tissue ablation, albeit a slightly larger layer of residual thermal damage. The larger residual thermal damage is likely responsible for its superior clinical effect in improving rhytids.


Fully ablative laser skin resurfacing leads to dramatic results ( Fig. 6.2 ), but also significant postoperative morbidity and complications. Ablation of the entire epidermis is essentially a well-controlled, partial-thickness skin burn. With this comes several postoperative days of copious oozing and crusting. Even with proper wound care, days to weeks off work and social activity are often required. During this time there is also significant infection risk. Once reepithelialization has occurred, the patients often experience prolonged erythema that can last 3 to 6 months. Even with experienced laser operators, the risk of scarring, permanent pigmentary changes, and demarcation lines with traditional resurfacing remain high. These adverse effects lead many to explore alternative methods to address photoaging.


To overcome the multitude of complications with traditional ablative resurfacing, nonablative technologies were developed. The goal of these devices was to target the tissue water within the dermis, resulting in thermal damage, protein denaturation, and subsequent collagen synthesis and tissue remodeling. A theoretical advantage of keeping the epidermis intact would result in a lower incidence of adverse events, namely infection, pigmentary alteration, and scarring. A variety of wavelengths were developed, including 1320- to 1450-nm devices. Indeed, any device that emits light absorbed by water and that repeatedly deposits heat in the skin does provide some degree of nonablative rejuvenation. This is also true for other nonablative devices classically used to treat superficial dyschromia and telangiectasias including vascular selective lasers, such as the pulsed-dye laser, and broad-band light sources . While the side effects and complications are significantly lower with nonablative devices compared with classical ablative lasers, so are the results.
Fractional Resurfacing
A paradigm shift with laser resurfacing occurred with the introduction of fractional photothermolysis . The concept was pioneered by Anderson and Manstein, and led to the first marketed device in 2004. Fractional photothermolysis uses narrow beams of high-energy light applied to the skin in a pixelated pattern. The tiny zones of treatment, termed “microthermal zones” (MTZs), are essentially islands of thermally damaged skin surrounded by normal tissue, allowing for rapid recovery from the uninjured reservoir. Fractionation can be applied to both ablative and nonablative lasers.
Since its introduction, fractional photothermolysis has been widely accepted as the first-line approach for most patients undergoing resurfacing. With the later introduction of ablative fractional resurfacing (AFR), combining features of classical ablative lasers and nonablative fractional photothermolysis, the armamentarium is even more equipped to deal with extrinsic and intrinsic aging. The remainder of this chapter will focus on fractional resurfacing, including clinical applications, patient selection, and potential complications.
Nonablative fractional resurfacing (NAFR) received clearance by the Food and Drug Administration in 2004 for the treatment of periorbital rhytids. Since then, numerous studies have demonstrated efficacy not only for rhytids but also for other indications, including dyschromia and acne scarring .
Central to treatment of all these conditions is the mechanism of action of NAFR. Various devices with wavelengths in the near-infrared spectrum (1400–1600 nm) are available from several manufacturers. The wavelengths of light emitted by these devices are significantly absorbed by water. The energy delivered causes zones of thermal coagulation within the dermis and a portion of the epidermis while leaving the stratum corneum intact, thus termed nonablative ( Fig. 6.3 ). The array, width, and density of these columns are dependent on both the device and parameters chosen.
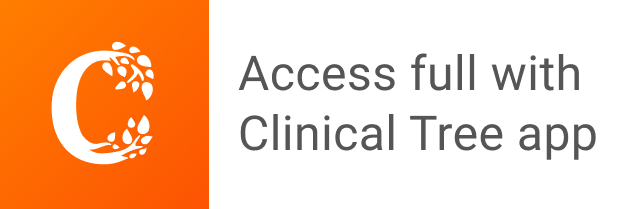