Lasers and Light Devices in Scar Management
Edward Victor Ross
James Daniel Jensen
KEY POINTS
Scar treatment with lasers should be guided by the features of the scar and the respective mechanisms of action of available technologies.
Typical scar reduction requires multiple treatments, potentially with multiple modalities; slow incremental improvement is the rule.
Laser treatment is often combined with injectable antimetabolites or corticosteroids to optimize outcomes.
Almost everyone has a scar, and although the prevalence of scars remains relatively unchanged, lasers and light sources have advanced their treatment. The foundation of the proper management of scars with lasers is an understanding of laser-tissue interactions. The outcome of these interventions depends both on the immediate laser-tissue interaction and on the cascade of wound healing events that follow.
The pathogenesis and wound healing aspects of scars are examined in other sections of the book (see Chapters 6, 9, and 10). In brief, at least in the case of a scar that begins as an open wound, early reepithelialization is the best predictor of the behavior of the scar over the next 6 months. Longer reepithelialization times, typically associated with deeper injures or those located on “underprivileged” skin (i.e., neck, upper chest, legs—almost any region off the face), are associated with a greater risk of hypertrophic or atrophic scarring.
In this chapter, we will concentrate on “open wound” scars as opposed to wounds that are closed primarily. However, the same principles may be applied to suboptimal scars resulting from surgical procedures. Once the skin has reepithelialized, typically occurring over a period of 1 to 4 weeks, a role for laser technology is most commonly considered. Not every scar requires an intervention, and the treating physician must consider which scars would benefit from laser treatment versus those that will benefit from tincture of time alone. Most studies of the role of lasers in scar reduction rely on nonobjective findings, and a healthy amount of skepticism should be directed toward publications or other observations that rely solely on visual improvements. The natural tendency for most scars is typically one of progressive gradual improvement. Therefore, the role of lasers is potentially twofold: (1) accelerate the spontaneous improvement in color, tone, and texture and (2) improve the ultimate form and function of scars that would otherwise not be observed in the natural course of healing. Another role for devices in scar management is “prevention.” Some studies have reported that laser procedures performed at the time of surgery or just before surgery can modulate the wound healing process.1,2 In some scenarios, this intervention can reduce the potential for adverse scarring.
Introduction to Lasers
Laser is an acronym for Light Amplification by Stimulated Emission of Radiation.3 Initially postulated by Albert Einstein in 1917 when he first introduced the concept of stimulated emission, the idea was enabled by the work of several scientists over the next four decades. The first viable laser device was demonstrated in 1960. Since that time, lasers have become available for numerous medical applications. In the early days of lasers, physicians feared that lasers, like ionizing radiation, might have some potential long-term effects on the skin such as mutagenicity and cancer. Fifty-five years later, these concerns have been largely dismissed.
Principles of Laser-Tissue Interactions
The laser operator should appreciate the fundamental principles of laser-tissue interactions. An understanding of how laser light interacts with the skin from a macroscopic and microscopic perspective allows the treating physician to optimize treatment results. Scar enhancement with lasers relies on a normalization of histology and a reduction in the contrast between scar tissue and adjacent unscarred skin.
Any light-based technology that modulates the behavior of a scar must take into account all of the potential opportunities to exploit the “contrast agents,” or chromophores, that differentiate the scar from normal skin.4,5,6,7 These contrast agents allow for selective heating of various components of the skin and, thus, selective modulation of the histology, appearance, and function of the scar.8 For example, when selectively targeting the red color in a scar, one applies wavelengths that selectively heat the blood vessels associated with the scar and avoids damaging the innocent bystander—in this case, the normal skin (Fig. 13-1).
Any light-based technology that modulates the behavior of a scar must take into account all of the potential opportunities to exploit the “contrast agents,” or chromophores, that differentiate the scar from normal skin.4,5,6,7 These contrast agents allow for selective heating of various components of the skin and, thus, selective modulation of the histology, appearance, and function of the scar.8 For example, when selectively targeting the red color in a scar, one applies wavelengths that selectively heat the blood vessels associated with the scar and avoids damaging the innocent bystander—in this case, the normal skin (Fig. 13-1).
Likewise, for treating a pigmented scar with a nonfractional technology, one might apply a wavelength that takes advantage of any pigment difference between the scar and surrounding skin.
There are several ways to limit heating with lasers to specific targets. In one scenario, using ubiquitous tissue water as the chromophore, laser “beams” are spatially confined by either using a microbeam with scanners or simply by having the operator, with the aid of magnifying loupes, use a small beam (0.2 to 2 mm) to precisely target a scar. An example is an exophytic scar related to acne, where the physician can precisely “whittle” the scar down, as one would knock down a stack of coins one by one. The other scenario is where the laser targets the scar based on the theory of selective photothermolysis (SPT), where wavelength and pulse duration direct heating only to areas with excess melanin or blood.5,9,10 According to this theory, through appropriate selection of pulse duration and wavelength, one can confine heat to a particular chromophore (i.e., pigment, hemoglobin [HgB], or water) within the scar. Shorter pulses generally are more likely to confine heat to the target, whereas longer pulses are less selective in heating. On the other hand, extremely short pulses can result in a very “violent” heating of the target (particularly in the case of Q-switched nanosecond or picosecond range lasers) where the subsequent inflammatory reaction can result in postinflammatory hyperpigmentation (PIH). This is of particular concern in darker skinned patients (see Chapter 18).11,12 Moreover, in the case of vascular scars, very short pulses can result in purpura; although purpura is sometimes a desirable reaction, in the setting of facial scar treatment purpura might limit social or work obligations.
The other primary determinant of selective heating is wavelength. In the absence of absorption—that is, in the absence of significant water, blood, or melanin absorption—longer wavelengths over the range of 400 to 2,000 nm penetrate deeper than shorter wavelengths. However, as wavelengths increase beyond 2,000 nm, the relative absorption of water increases, thereby limiting the depth of penetration (Fig. 13-2A,B). This phenomenon should be a consideration in proper selection of parameters for optimal scar management.
One can think of light interacting with the skin in a similar manner as with other optical phenomena, where there is a loss of a fraction of incident light from reflection and scattering upon contact with the skin surface. In short, upon initial impact of light on the surface, a portion of the incident light is reflected (about 5%), and the remainder enters into the skin just below the stratum corneum.7,13 Once the light has penetrated the skin surface, the skin’s optical properties determine the propagation of light.
In general, three effects guide the propagation of light in the skin (Fig. 13-3):
Reflection and refraction
Absorption
Scattering
For purposes of the skin, where the medium is opaque, refraction is hard to measure within the context of absorption and scattering. If we consider a slice of skin in cross section, only a portion of photons will be transmitted to the base of the slice. These are the nonreflected, nonabsorbed, and forward-scattered photons. When a surface is smooth, the reflection is said to be “specular,” and when it is rough, the reflection is said to be “diffuse.” As most tissue is rough, save for some wet surfaces, diffuse reflection typically exceeds specular reflection (Fig. 13-4). Refraction occurs when two media show different light velocities in their respective regions. This causes bending of the light at the interface (Fig. 13-5). Reflectance is the ratio of the intensities of reflected light to the intensity of the light propagating to the next layer. Reflectance depends on the angle of incidence, the polarization of the radiation, and indices of refraction of the two media at the boundary.
Generally, as the angle of incidence (the angle between the light ray and the direction perpendicular to the skin
surface) approaches 90 degrees, reflectance increases. The absorbance of a medium is defined as the ratio of the absorbed and incident intensities. Selective absorption is the absorption of certain wavelengths over others. Attenuation of the laser beam due to absorption is directed by Beer-Lambert law.
surface) approaches 90 degrees, reflectance increases. The absorbance of a medium is defined as the ratio of the absorbed and incident intensities. Selective absorption is the absorption of certain wavelengths over others. Attenuation of the laser beam due to absorption is directed by Beer-Lambert law.
![]() FIGURE 13-3 Figure showing the behavior of light at the skin interface. (From Figure 1 in Anderson RR, Parrish JA. The optics of human skin. J Investig Dermatol. 1981;77(1):13-19.) |
![]() FIGURE 13-4 Random path of photons in skin. (From Figure 2.3 in Welch AJ, Van Gemert MJ, eds. Optical-Thermal Response of Laser-Irradiated Tissue. New York, NY: Plenum Press; 1995.) |
This law states that the absorption “length” in the tissue is given by

where Z is the distance over which the intensity diminishes to 1/e of its incident “strength,” Iz is the intensity at the depth z, Io is the incident intensity, and α is the absorption coefficient.
The inverse of this coefficient is the absorption length, or

In the infrared (IR) region, water is the main absorber, whereas in the visual and ultraviolet (UV) portions of the spectrum, proteins and pigment are the primary absorbers. The two primary discrete absorbers in skin are HgB and melanin. Melanin is the main chromophore in the epidermis, and its absorption decreases almost linearly as a function of wavelength over 400 to 1,000 nm. HgB has
relative peaks at 280, 420, 540, and 580 nm, with a much smaller peak at 940 nm (Fig. 13-1). As neither water nor HgB absorbs strongly between 600 and 1,200 nm, there is a so-called therapeutic window in this range where light penetrates more deeply.7,13
relative peaks at 280, 420, 540, and 580 nm, with a much smaller peak at 940 nm (Fig. 13-1). As neither water nor HgB absorbs strongly between 600 and 1,200 nm, there is a so-called therapeutic window in this range where light penetrates more deeply.7,13
Absorption is related to specific wavelength-dependent bonds, where particles are set into motion by electromagnetic (EM) fields; the frequency of the wave makes for transitional (electron transition level changes in the shell chemical model) or vibrational changes in the target. With scattering, the frequencies do not match but rather result in forced vibration, causing photons to slow down in a denser medium.
Usually we consider a turbid medium (like the skin) as showing both absorption and scattering, and we can define two coefficients that characterize the attenuation of light in the skin (scattering and absorption coefficients). A good “phantom” for the dermis is milk, a turbid material that scatters light much like the skin. If one, for example, takes a laser pointer and applies it to the surface of a glass of milk, one will observe the linear beam spread just beneath the surface. There are many methods to analyze the nature of scattering in the skin, among them Monte Carlo models (where statistical models of chance characterize the migration of photons in the skin).14,15 One should note that the skin is heterogeneous and fragile, and many of the predicted behaviors of light and the skin are not reliably observed. Ultimately, the immediate end response is the best predictor of the therapeutic outcome for any laser tissue interaction,16,17 and this concept is especially true in scar management and rehabilitation.
The nonpigmented portion of the epidermis and the dermis serves as a scattering medium, whereas blood, melanin, and water contained in these layers act as the most significant chromophores (absorbers) within the skin. If one examines the absorbance of blood, melanin, and water along the EM spectrum shown in Figure 13-1, blood and melanin absorption is most prominent along the 400 to 800 nm portion of the EM spectrum, whereas water has little absorbance within this range. The absorbance of water, however, increases significantly in the IR portion of the EM spectrum, with an absorption peak at 2,940 nm (Fig. 13-2A).
In any laser-tissue interaction, the local peak elevation in temperature is characterized by the following equation:

where Fz is the local subsurface fluence, ρ is the density, c is the specific heat, g is a geometric factor (“1” for planes, “2” for cylinders, and “3” for spheres), τp is the laser pulse duration, and τr is the thermal relaxation time of the target (time for target to cool to 37% of peak temperature), defined by:

where D is the thickness or diameter of the target, g is the geometric factor referenced earlier, and K is the thermal diffusivity (a measure that includes the heat capacity and thermal conductivity of the material). For example, a potato would show a relatively low thermal diffusivity because of its low thermal conductivity and high specific heat, whereas a small metal tattoo particle would show a high thermal diffusivity.
Fz is determined largely by the attenuation by scattering and absorption. The probabilities of absorption or scattering (designated µa and µs, respectively) are determined by experiment. For example, for a µa of 0.3 per cm, the mean free path before absorption is 1/µa, or 3.3 cm. Generally, light is attenuated as it propagates through tissue. In turbid tissue (i.e., the dermis, where collagen acts as the major scatterer), the fluence attenuation can be described by:

where Fo is the incident fluence, F(z) is the local subsurface fluence at some depth z, k is a constant that accounts for backscattered light, and δ is the wavelength-dependent optical penetration depth of light, or the depth at which there is attenuation to 37% of the surface value (37% = 1/e, where e = 2.7, the base of the natural logarithm). This depth is determined by absorption and scattering coefficients, as related by the simple equation below:

Blood
The greatest practical absorption peaks for blood are in the green and yellow spectral regions. Most of the blood in the skin is in the form of oxygenated HgB; however, approximately 30% is in the deoxygenated state, and a still smaller fraction is in the methemoglobin state.18
Recent work has highlighted the optimal wavelengths for targeting venous versus arterial blood (primarily mirroring the relative fractions of oxygenated and deoxygenated HgB). However, the optimal wavelengths for targeting venous versus arterial blood also have overall lower associated blood absorption coefficients. For example, a much lower fluence would be required to generate reduction of erythema using green-yellow light wavelengths (e.g., 532 and 595 nm) than, for example, the alexandrite laser (755 nm, near-IR spectrum, preferentially targeting deoxygenated HgB), where very large fluences must be delivered to achieve a similar effect.19
Melanin
Melanin has a broad absorption spectrum. This broad absorption helps to protect the skin from harmful radiation from the sun. In patients with a lighter skin phenotype (i.e., Fitzpatrick type I, II), pigment lying within a scar is easily targeted because of the relative lack of pigment in the surrounding, normal skin. In darker skin types, however, the laser operator should take care not to overtreat pigment within a scar and cause further dyspigmentation (i.e., hypopigmentation). The broad absorption spectrum of melanin is a double-edged sword. On one hand, the broad absorption allows many types of lasers to target pigment within the skin, thus giving the laser operator many choices for treating unwanted melanin deposition. On the other hand, many lasers that are intended to target other chromophores (i.e., blood) can also unintentionally be absorbed
by melanin.20 Usually, melanin is found in the epidermis superficial to the excess blood (with the exception of some scars with excess dermal melanin—usually long-standing scars or acne scars associated with medication-induced dyspigmentation, such as from the drug minocycline). It follows that treating a red scar in dark skin is a challenge. This is because melanin has a broad and relatively nonspecific spectrum of absorption, and will absorb the energy from a laser directed toward blood. Consequently, if a patient with Fitzpatrick type IV skin were to present for treatment of hyperemia associated with a thyroid scar, the operator must reduce settings to avoid overtreatment of the epidermis. Fortunately, however, the same melanin that poses such a concern for the laser operator also tends to mask underlying hyperemia.21,22,23
by melanin.20 Usually, melanin is found in the epidermis superficial to the excess blood (with the exception of some scars with excess dermal melanin—usually long-standing scars or acne scars associated with medication-induced dyspigmentation, such as from the drug minocycline). It follows that treating a red scar in dark skin is a challenge. This is because melanin has a broad and relatively nonspecific spectrum of absorption, and will absorb the energy from a laser directed toward blood. Consequently, if a patient with Fitzpatrick type IV skin were to present for treatment of hyperemia associated with a thyroid scar, the operator must reduce settings to avoid overtreatment of the epidermis. Fortunately, however, the same melanin that poses such a concern for the laser operator also tends to mask underlying hyperemia.21,22,23
Water
Water is ubiquitous in the skin, and wavelength ranges from 1,200 to 2,000 nm show intermediate absorption, whereas 2,940 nm (erbium:yttrium-aluminum-garnet—Er:YAG laser output) and 10,600 nm (carbon dioxide—CO2 laser output) show very strong absorption. It follows that the former wavelengths are associated with nonablative or semi-ablative treatments, and the latter with purely ablative treatments. Both ablative and nonablative wavelengths are applied with scanners that can deliver the energy in a fractional manner, meaning that a grid-like pattern of columns of controlled tissue damage may be reliably produced (Fig. 13-6).24,25,26,27 Through proper selection of wavelength, pulse duration, and microspot diameter (normally between 100 and 430 µm), a particular cross-sectional density and depth of wounds can be created. The typical geometry of the wounds is cylindrical craters. However, other manufacturers have designed lasers that create a cross-hatched grid pattern of microinjuries. Radiofrequency (RF) needle devices can also create controlled thermal injuries in a fractionated pattern; deeper injuries with relative epidermal sparing can be achieved through the use of insulated needles.28
Interaction Types
Most laser-tissue interactions are thermal in nature. Other potential mechanisms are photochemical (e.g., photodynamic therapy, PDT) and plasma-induced ablation (termed photoablation or photodisruption) (Fig. 13-7). Photodisruption is a form of minimally invasive surgery used in ophthalmology; IR neodymium (Nd):YAG lasers form a plasma (“lightning bolt”), which then causes acoustic shock waves (like a “thunderclap”), which in turn affects tissue.
Photochemical
PDT has been used in the management of some scars. In one study Chang et al.29 examined aminolevulinic acid (ALA) PDT in scars and the mechanism of action. They found that for fibroblast cell cultures from hypertrophic scars, apoptosis was observed through a p53-associated signaling pathway. Challenges in PDT include (a) achieving sufficient accumulation of ALA in fibroblasts versus the epidermis and (b) pain management. Another obstacle is penetration of the light, as the presumed fibroblast targets reside up to several millimeters deep in the dermis for thicker scars.29,30
Another type of intervention is biostimulation (aka low-level light therapy, LLLT), where presumably certain frequencies of light can modify mitochondrial behavior through the cytochrome system. For example, Freitas et al.31 showed that LLLT works by interacting with the cytochrome system in cells, such that changes in adenosine triphosphate and
interleukin 6 expression have been noted. LLLT usually involves wavelengths between 600 and 1,100 nm so that adequate penetration depth is achieved. In one study 800 nm light at 500 mW was applied at 1 W per cm2 for a total of 4 J per cm2, three times per week for 5 weeks. Overall, they found no significant differences between placebo and experimental groups evaluated using the Vancouver Scar Scale (VSS). However, tendencies toward greater improvement in the treatment group were found.31
interleukin 6 expression have been noted. LLLT usually involves wavelengths between 600 and 1,100 nm so that adequate penetration depth is achieved. In one study 800 nm light at 500 mW was applied at 1 W per cm2 for a total of 4 J per cm2, three times per week for 5 weeks. Overall, they found no significant differences between placebo and experimental groups evaluated using the Vancouver Scar Scale (VSS). However, tendencies toward greater improvement in the treatment group were found.31
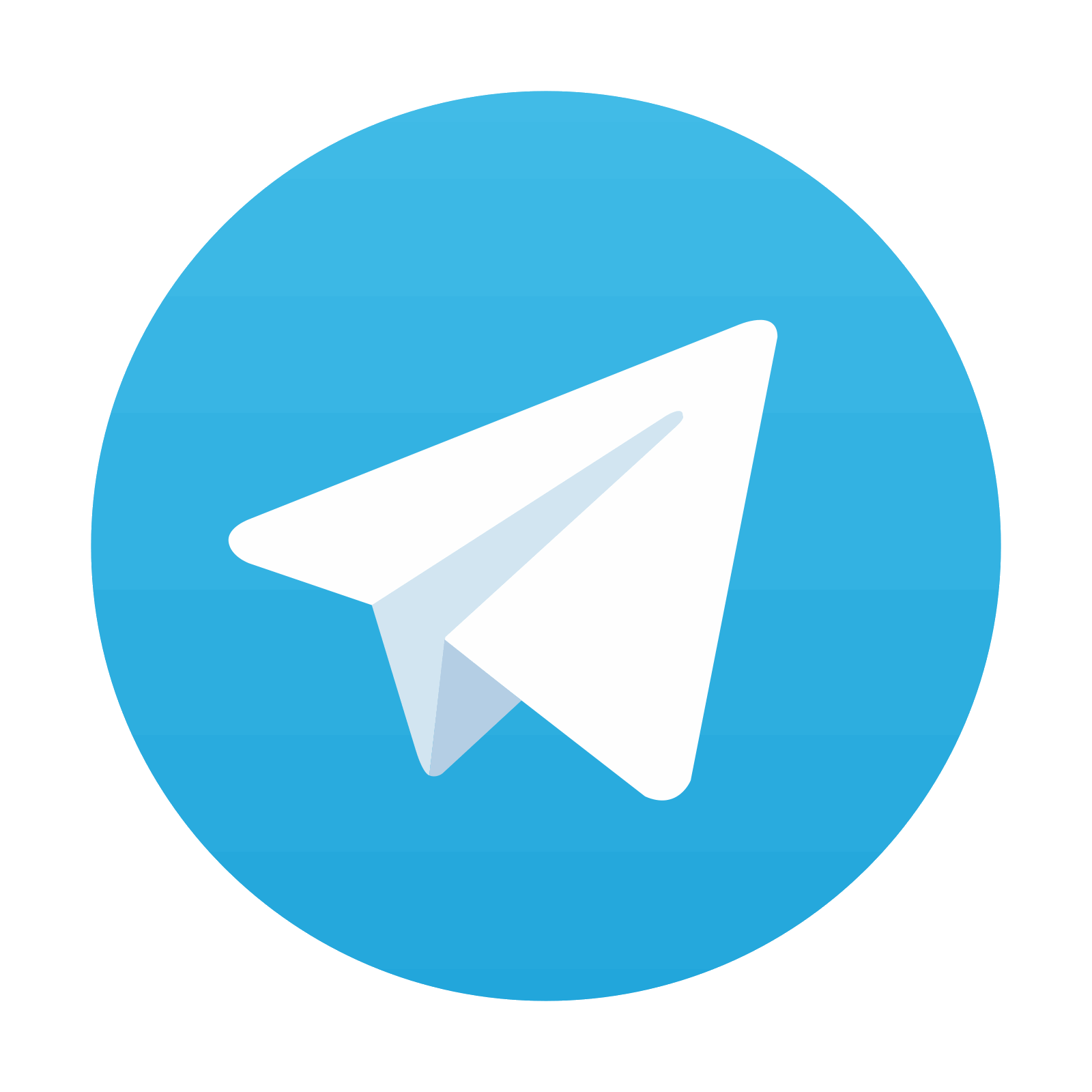
Stay updated, free articles. Join our Telegram channel
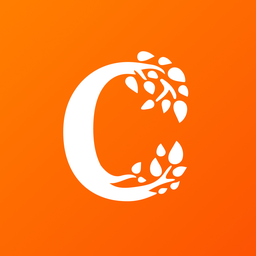
Full access? Get Clinical Tree
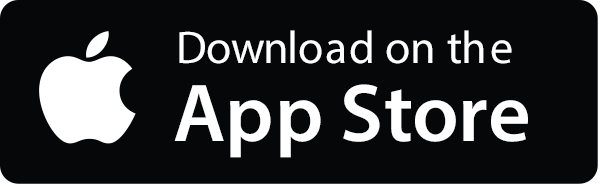
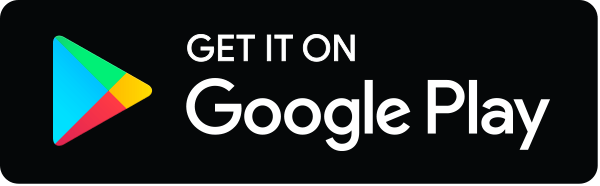