Abstract
The blood vessels of the skin are involved in the control of body temperature and they provide a conduit for the supply of nutrients and oxygen to the skin as well as the rapid disposal of metabolic waste products. Recent scientific progress in the field of vascular biology has provided ample evidence that blood vessels and lymphatic vessels also play essential roles in: (1) the development and progression of skin tumors; (2) the mediation and perpetuation of cutaneous inflammation; and (3) physiologic processes such as tissue repair and hair follicle growth. An increased knowledge regarding markers for specific types of vessels, together with the identification of key molecules that control vascular growth and function, has led to an in-depth understanding of the mechanisms that control the function of the cutaneous vascular system in health and disease. As a result, there are important implications for the development of novel therapeutic strategies for the treatment of skin diseases.
Keywords
vascular system, blood vessels, endothelial cells, lymphatic vessels, angiogenesis, lymphangiogenesis, VEGF-A, VEGFR-3, cutaneous blood vessels, tip cells, stalk cells
- ▪
Cutaneous blood vessels and lymphatic vessels play important roles in skin inflammation and tumor progression
- ▪
Vascular endothelial growth factor-A (VEGF-A) and placental growth factor (PlGF) are major skin angiogenesis factors
- ▪
Expression of VEGF and PlGF by keratinocytes is upregulated in psoriasis, healing wounds, and cutaneous squamous cell carcinomas
- ▪
VEGF-C and VEGF-D induce skin lymphangiogenesis via interaction with VEGF receptor-3 on lymphatic endothelium
- ▪
Mutations in several genes, including FLT4 which encodes VEGF receptor-3, have been identified in congenital lymphedema
- ▪
Blood and lymphatic vessels represent new targets for anticancer and anti-inflammatory therapies
Structure and Function of Cutaneous Blood Vessels
Blood vessels are essential in order to: supply sufficient oxygen and nutrients to the skin, maintain normal tissue homeostasis and function, and meet the increased nutritional needs of the skin in various pathologic conditions. Blood flows from arteries and arterioles through capillary loops to postcapillary venules and veins. For practical and didactic purposes, the vascular system of the skin has been divided into a superficial and a deep vascular plexus ( Fig. 102.1 ), with additional vascular networks surrounding sweat glands and hair follicles.

The architecture of the cutaneous vascular system varies in different areas of the body. In the presence of pronounced epidermal rete ridges, such as in the skin overlying the extensor aspects of the joints or on the palms and soles, straight capillary loops extend into the elongated dermal papillae. In contrast, rete ridge formation and papillary loop formation are less pronounced in sites such as the abdominal skin. The shape of the papillary microvessels exactly reflects the three-dimensional architecture of the epidermal rete ridges, typically with one or two loops within each dermal papilla . The inner diameter of papillary blood vessels averages between 5 and 10 microns. In contrast to the idealized architectural model of the cutaneous vascular system, vascular perfusion studies ( Fig. 102.2 ) and light-sheet fluorescence microscopy studies ( Fig. 102.3 ) revealed a rather continuous, sometimes irregular meshwork of interconnecting cutaneous blood vessels. Consistently, blood vessels are found immediately below the non-vascularized epidermis.


Anagen hair follicles are highly vascularized. Immunohistochemical and perfusion studies suggest that the frequently single blood vessel within the mesenchymal hair papilla is derived from the lower vascular plexus, whereas the dense vascular network surrounding the hair follicle is predominantly derived from the upper vascular plexus ( Fig. 102.4 ). The relatively small blood vessels that are found during the telogen resting phase elongate and enlarge during the anagen growth phase, and then they extend towards the subcutis together with the growing hair follicle . During the catagen phase, hair follicle involution is associated with regression of perifollicular blood vessels and with increased rates of endothelial cell apoptosis. The extent of perifollicular vascularization may directly influence the size of the hair follicles .

The cutaneous vasculature also plays a systemic role in regulating body temperature and blood pressure. Arterioles possess precapillary muscular sphincters that, upon contraction, shunt the blood via arteriovenous anastomoses directly to venules, thereby bypassing the capillary networks. Arteriovenous anastomoses are frequently found in the fingertips, where they form the glomi or glomus bodies . Glomi have autonomous innervation and control peripheral blood flow via modulation of their muscular contraction levels.
All blood vessels contain a continuous inner monolayer of flat endothelial cells that are surrounded, on the external side, by a continuous basement membrane. Smaller vessels contain a second, sometimes discontinuous, layer of perivascular cells called pericytes, which are surrounded by a basement membrane that is continuous with the endothelial basement membrane. Pericytes play an important role in the maintenance of mature blood vessels, and detachment of pericytes from endothelial cells is an early event during sprouting angiogenesis (outgrowth of new capillaries from pre-existing blood vessels). Arterioles and venules are also surrounded by pericytes, and these cells can be visualized by staining for platelet-derived growth factor receptor-β (PDGFR-β), NG2 (chondroitin sulfate proteoglycan 4), α-smooth muscle actin, and desmin. The walls of most arterioles and larger venules and veins contain contractile smooth muscle cells that are surrounded by a basement membrane. Vascular basement membranes contain collagen types IV, XV and XVIII, laminin, fibronectin and other extracellular matrix proteins (see Ch. 95 ).
At the ultrastructural level, vascular endothelial cells are characterized by tight junctions between neighboring cells and by specialized organelles, the rod-shaped Weibel–Palade bodies ( Fig. 102.5 ), that serve as storage organelles for the coagulation factor von Willebrand factor, also known as factor VIII-related antigen. Weibel–Palade bodies also contain P-selectin, angiopoietin-2, and endothelin-1. Activation of endothelial cells by proinflammatory cytokines or pro-angiogenic factors readily leads to translocation of these storage organelles to the cell membrane, with consecutive enhanced membrane expression of P-selectin and release of von Willebrand factor. Other characteristic ultrastructural features include caveolae (minute invaginations of the membrane surface), pinocytotic vesicles, and the formation of vesiculo-vacuolar organelles (VVOs) . Circulating macromolecules cross the endothelium through interendothelial cell gaps and transendothelial cell pores, some of which arise from VVOs. Endothelial cell fenestrations, areas with direct apposition of the endothelial cell membranes without intervening cytoplasm, are rarely seen in normal skin except in angiogenic perifollicular blood vessels during the growth phase of the hair follicle. However, fenestrated endothelial cells are frequently seen in skin diseases with pronounced angiogenesis and vascular hyperpermeability, including psoriasis.

Several markers are utilized for immunohistochemical staining of blood vascular endothelial cells ( Table 102.1 ). In normal human skin, the most specific markers for cutaneous blood vessels appear to be von Willebrand factor (see Fig. 102.3 ), CD34, and PV-1 (plasmalemma vesicle-associated protein-1); the latter is recognized by the PAL-E (Pathologische Anatomie Leiden-Endothelium) antibody. Double immunofluorescence stains for the pan-endothelial cell marker CD31 (PECAM-1) plus the lymphatic vessel endothelium-specific hyaluronan receptor LYVE-1 or the glycoprotein podoplanin (recognized by the widely used D2-40 antibody) also allow for the selective visualization of LYVE-1-negative (or podoplanin-negative), CD31-positive blood vessels in the skin. As a result, blood vessels can be distinguished from lymphatic vessels (see Fig. 102.15 ).

Vascular Development
During embryogenesis, the first blood vessels are formed through vasculogenesis , the differentiation of undifferentiated angioblasts into endothelial cells that form a primitive vascular network. Angioblasts originate from blood islands in the extraembryonic mesoderm of the yolk sac. Blood islands are clusters of epithelioid cells; the outer cells differentiate into angioblasts, whereas the inner cells differentiate into primitive hematopoietic cells, suggesting that both cell types originate from a common embryonic precursor cell, the hemangioblast; the latter is formed from earlier precursor cells under the influence of fibroblast growth factors (FGFs) ( Fig. 102.6 ). Hemangioblasts already express the high-affinity receptor for vascular endothelial growth factor-A (VEGF-A), vascular endothelial growth factor receptor-2 (VEGFR-2; KDR [human]; Flk-1 [murine]).

Angioblasts develop under the influence of stimulation by VEGF-A and express several vascular endothelial cell markers, including the Tie-1 and Tie-2 receptors, vascular endothelial (VE)-cadherin, and the high-affinity VEGF receptors 1 (Flt-1), 2 (KDR), and 3 (Flt-4). Angioblasts within the embryo appear along the anterior intestinal portal and along the lateral edges of the somites, later coalescing to form the dorsal aorta and the large vessel primordia of the body. Angioblasts are highly migratory “prevascular” endothelial cells and are found throughout the embryo with the exception of tissues such as cartilage and epithelia.
When angioblasts form a lumen, they become endothelial cells with a polarized phenotype and production of a basal lamina. Interactions of integrin receptors on the endothelial surface with the basement membrane are of paramount importance for the formation of the primordial vascular plexus, and establishment of interendothelial adherence junctions requires the vascular endothelial (VE)-cadherin. The early vascular system consists of sinusoidal capillaries that are arranged in a polygonal honeycomb pattern (see Fig. 102.6 ). The primordial vascular plexus then becomes surrounded by mesenchymal cells that give rise to pericytes and vascular smooth muscle cells. In a next step, under the influence of VEGF-A, angiopoietins and ephrins, profound remodeling of the primordial vascular plexus takes place (see below). Remodeling involves both the formation and the regression of blood vessels and apoptosis of vascular endothelial cells.
Angiogenesis , the sprouting of new blood vessels from existing primordial vessels, represents the major mechanism for new blood vessel formation . During this process, specialized endothelial cells, so-called tip cells, extend filopodia and sense the environment for guidance cues such as VEGF-A ( Fig. 102.7 ). Tip cells are followed by stalk cells which extend the sprout by proliferation and form a lumen . Tip cells can connect with neighboring tip cells to form new vascular branches . The specification of tip and stalk cells is regulated by Notch signaling. Activation of VEGFR-2 on tip cells by VEGF-A results in increased expression of the Notch ligand Delta-like 4 (Dll4); the latter binds to Notch expressed on neighboring stalk cells. Notch signaling in stalk cells leads to reduced VEGFR-2 expression, rendering these cells less responsive to VEGF-A (see Fig. 102.7 ). This is a highly dynamic process in which endothelial cells compete for the tip cell position . Recent findings have shown that glycolysis plays an additional important role in the regulation of vascular sprouting, in parallel with genetic cues .

The Tie-2 receptor tyrosine kinase, expressed on vascular endothelial cells, also plays a crucial role in vascular sprouting and remodeling during early embryonic angiogenesis. Pericyte-derived angiopoietin-1 (Ang-1) activates the Tie-2 receptor, whereas Ang-2 can act as an inhibitor of Ang-1 under angiogenic conditions ( Fig. 102.8 ). In addition, Eph–ephrin receptor interactions, via bidirectional signaling, play an important role in vascular remodeling and in the determination of vascular identity. Ephrin B2 is specifically expressed by arterial endothelium, whereas its receptor, Eph-B4, is expressed by venous endothelium. Both molecules are involved in the definition of boundaries between arterial and venous endothelial cells.

Additional molecules are involved in the control of embryonic angiogenesis and vascular remodeling, including semaphorins, transforming growth factor-β (TGF-β), endoglin (the low-affinity receptor for TGF-β), and activin receptor-like kinase-1. Of note, dysfunction of the latter two proteins is seen in hereditary hemorrhagic telangiectasia (see below). Platelet-derived growth factor subunit B (PDGFB), secreted by endothelial cells, plays a critical role in the recruitment and differentiation of pericytes and periendothelial smooth muscle cells that contribute to the stability of mature blood vessels .
Regulation of Skin Angiogenesis
Angiogenesis is a common feature of developing skin during embryogenesis. In contrast, the blood vessels in healthy adult skin are predominantly quiescent, with the exception of the expansion and involution of perifollicular blood vessels during the hair cycle . However, adult skin retains the capacity for brisk initiation of angiogenesis during wound healing, in the setting of inflammation, and during neoplastic growth. Angiogenesis occurs as: (1) the sprouting outgrowth of new capillaries from pre-existing postcapillary venules; or (2) non-sprouting remodeling of pre-existing blood vessels either through circumferential growth/vascular enlargement or through the formation of intravascular endothelial cell pillars (intussusception). Angiogenesis is a multistep process that often involves as a first step the induction of microvascular hyperpermeability, i.e. increased vascular leakage, leading to extravasation of plasma proteins such as fibrinogen and prothrombin ( Table 102.2 ). Fibrinogen is then further processed to fibrin and serves as a provisional matrix for migrating endothelial cells, whereas the generation of thrombin acts as an initiator of the extrinsic coagulation cascade, leading to cleavage and activation of several matrix proteins . Together, these processes lead to the generation of a highly angiogenic stroma.
THE STEPWISE INDUCTION OF ANGIOGENESIS |
|
A major function of pericytes appears to be the maintenance of mature and quiescent blood vessels (a function which is interrupted during angiogenesis). In normal adult skin, pericytes secrete Ang-1, which leads to phosphorylation of the Tie-2 receptor (a receptor exclusively expressed on endothelial cells) and maintenance of the mature vascular architecture with low vascular permeability (see Fig. 102.8 ). In turn, endothelial cells secrete growth factors that mediate the recruitment of pericytes, including PDGFB that binds to the PDGFR-β on pericytes. During angiogenesis, endothelial cells express high amounts of Ang-2, a functional antagonist of Ang-1 in activated endothelium, thereby blocking the maturation-inducing effect of Ang-1 and leading to detachment of pericytes from vascular endothelial cells . In the presence of VEGF-A, endothelial cells then undergo the consecutive steps of angiogenesis, whereas in the absence of VEGF-A, vascular endothelial cells undergo apoptosis (see Fig. 102.8 ). Both VEGF-A and Ang-2 expression are upregulated in angiogenic skin diseases, including psoriasis, and keratinocyte-derived VEGF-A and placental growth factor (PlGF; see below) both potently induce the expression of Ang-2 in dermal microvascular endothelial cells. There is evidence that bone marrow-derived progenitor cells also contribute to the angiogenic response. Most of these cells differentiate into perivascular macrophages that secrete angiogenic factors including VEGF-A.
The vascular basement membrane serves as a natural barrier that prevents sprouting of endothelial cells from quiescent vessels, with pericytes assisting in the maintenance of the quiescent state. An early step in the angiogenic response involves the degradation of vascular basement membrane components such as collagen type IV through the concerted action of activated proteases, including matrix metalloproteinase (MMP)-2 and MMP-9 . Integrin receptors on the surface of activated endothelial cells, in particular the α 1 β 1 , α 2 β 1 and α v β 3 integrins, then interact with either native or degraded matrix molecules (e.g. collagen type I, fibronectin) in order to facilitate endothelial cell migration . The migration of endothelial cells in the skin is directed towards a gradient of angiogenesis factors that are most commonly produced by the epidermis, but that can also be secreted by macrophages and other stromal cells. Endothelial cells then undergo a series of cell divisions, promoted by potent angiogenesis factors such as VEGF-A and basic fibroblast growth factor (bFGF). Finally, endothelial cells form vascular lumina and mature new blood vessels with basement membrane and pericyte coverage.
In normal skin, vascular quiescence is maintained by the dominant influence of potent endogenous angiogenesis inhibitors over angiogenic stimuli, whereas angiogenesis is induced by increased secretion of angiogenic factors and/or by down-regulation of angiogenesis inhibitors. Several factors that control angiogenesis within the skin have been identified. The major skin angiogenesis factor, VEGF-A, was originally discovered as vascular permeability factor (VPF), due to the activity of tumor cell-conditioned media to induce accumulation of ascites fluid . VEGF-A is a homodimeric, heparin-binding glycoprotein occurring in at least four isoforms of 121, 165, 189 and 201 amino acids in human skin, due to alternative splicing. VEGF-A binds to two type III tyrosine kinase receptors that are expressed predominantly on vascular endothelial cells, Flt-1/VEGF receptor-1 (VEGFR-1) and KDR/Flk-1/VEGFR-2 ( Fig. 102.9 ). In addition, VEGF-A165 also binds to the neuropilin-1 receptor on endothelial and other cells.
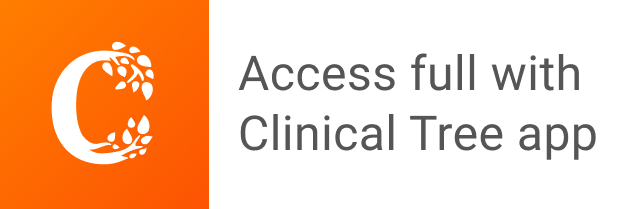