Scar Treatment, Restoration, and Prevention—Beyond the Horizon?
Kachiu C. Lee
R. Rox Anderson
KEY POINTS
Nature creates new tissue through three major pathways: tissue genesis, remodeling, and scarring.
Scar mitigation and prevention after major injury relies on finding cellular and molecular strategies to guide the wound healing process toward the remodeling and regenerative pathways. Recent advances in our understanding of wound healing and scarring processes offer the promise of better treatments in the future, with several approaches demonstrating improvements in scar quality.
The novel concept of “tissue copying” exemplifies these principles, facilitating the healing of large-scale wounds with the creation of thousands of microscopic-scale wounds that heal scarlessly through remodeling.
This book exposes the awesome human suffering that scarring can pose; it summarizes both our current understanding and how little we really know. This chapter is an attempt to synthesize many viewpoints by guessing about the future. Is suppression of scarring possible? If so, is there an underlying ability to heal by tissue regeneration or tissue remodeling? How far along are we now? What are the most promising strategies for medical, cellular, and/or surgical treatments? Are new technologies on the horizon? How far-reaching would a “cure” for scarring really be? Questions and possibilities, not answers, are the stuff of this chapter.
Scarring is a concerted response to gross injury that evolved long ago among vertebrates, but recently in the history of all living things on earth. Scarring confers a survival advantage—or at least it used to, before medical care was invented. Meanwhile, all creatures (including ourselves) can heal micro-wounds without scarring. Surprisingly, even scar tissue retains at least some of that ability. After micro-wounding (such as skin needling or fractional laser therapy), scars normalize to some extent (see Chapters 5 and 13). The emergence of scarring appears to be linked to the emergence of adaptive immunity, both during evolution and in an individual human fetus during its first and second trimesters (see Chapter 27). Even in adult mammals, there are many examples of tissue regeneration without scarring—all of which rein in a full-blown immune response—through systemic or local tissue mechanisms. Scars are an example of tissue with sustained inflammation. They are composed of abnormal connective tissue loaded with mast cells, inflammatory mediators, neovascularization, and lifelong hyperactive turnover of the extracellular matrix. Why? How? Thanks to the tools of molecular biology, our basic understanding of scarring has improved dramatically of late. That knowledge will eventually be the basis for specific, new molecular and cellular strategies to prevent, treat, or even fully reverse scarring.
New Wound Treatments
The missing, dead, or dying tissue of a major wound is not doomed to be replaced by a scar. Prevention of scarring might be as simple as making sure that appropriate, normal skin tissue replaces the major wound defect. That is the essence of full-thickness skin grafting, which immediately closes a wound, often with excellent results. Unfortunately, full-thickness grafting creates another full-thickness skin wound at the donor site, which limits this strategy to small wounds. Also, full-thickness skin grafts may or may not “take,” which is equivalent to saying that they may or may not connect with the wound bed’s underlying blood supply before the graft tissue dies. Even so, a successful full-thickness skin graft is nothing short of miraculous. First, it is miraculous that full-thickness skin can stay alive for days near body temperature without any blood supply (what can we do to extend that time?). Second, it is miraculous that new connections form so rapidly between vessels in the graft and vessels in the underlying wound bed (how can we enhance that process?).
For extensive wounds, split-thickness skin grafts are preferred because they can easily be expanded to cover a larger area and because the donor site eventually heals with a new epidermis formed from stem cells residing in hair follicles. Unfortunately, a disfiguring and often painful scar typically results at the donor site. As such, split-thickness
skin graft donor sites are an excellent setting to test new scar prevention strategies. Unfortunately, split-thickness skin grafts are only a thin, superficial layer of epidermis and dermis—not fully functional skin. Hair follicles, sweat glands, reticular dermis, subcutaneous fat, and other deep structures are missing from split-thickness grafts. Less than a few hundred micrometers thick, split-thickness grafts can survive longer than full-thickness grafts without a blood supply. In essence, thin pieces of tissue can maintain viability by diffusion of oxygen and nutrients, until a blood supply arrives.
skin graft donor sites are an excellent setting to test new scar prevention strategies. Unfortunately, split-thickness skin grafts are only a thin, superficial layer of epidermis and dermis—not fully functional skin. Hair follicles, sweat glands, reticular dermis, subcutaneous fat, and other deep structures are missing from split-thickness grafts. Less than a few hundred micrometers thick, split-thickness grafts can survive longer than full-thickness grafts without a blood supply. In essence, thin pieces of tissue can maintain viability by diffusion of oxygen and nutrients, until a blood supply arrives.
If the signals that initiate and sustain scarring were blocked, would regeneration take over? Does regeneration require its own specific set of signals? These questions have been answered in part. For example, members of the transforming growth factor beta (TGF-β) family of proteins have been identified as playing major roles in scar initiation. TGF-β3 in particular can promote scarless wound healing in some mammals, whereas TGF-β1 and TGF-β2 are early signals that tend to drive scar formation. Corticosteroids, which have been used for decades to control the growth of hypertrophic scars, typically increase TGF-β3 while decreasing TGF-β1 and TGF-β2.1,2,3,4 Immediately after wounding, a sequence of cellular and molecular events occur that set forth a course of healing (see Chapter 6). In the dermis, there is a large reservoir of preformed TGFs bound to collagen, which are rapidly released and activated upon injury. An immediate medical therapy aimed at “sopping up” proscarring signals, while adding antiscarring signals, might reduce or eliminate the scarring pathway. Other potent signals derive from platelet activation, a nearly universal event after wounding. Later in the course of scar tissue formation, other growth factors from platelets appear to improve the scar. We can look forward to specific new drugs that influence the molecular pathways involved in scarring.
One fundamental goal in the field of tissue engineering is to create fully functional skin or other organs ex vivo, which can then be used for transplantation. This lofty goal amounts to mimicking or recapitulating organogenesis, without a body. For decades since the discovery that a fully differentiated epidermis could be grown from keratinocytes atop a feeder layer of cultured fibroblasts, there has been steady progress in de novo skin engineering. Autologous keratinocytes were initially used, followed by several commercial products using allogeneic cells.5 Even though eventually replaced by the host, putting this “ersatz” allogeneic version of a split-thickness skin graft onto a clean wound bed enhances healing and, to some extent, minimizes scarring. Until tissue engineers figure out how to create full-thickness, autologous, fully functional skin in culture, this fundamental goal will remain elusive.
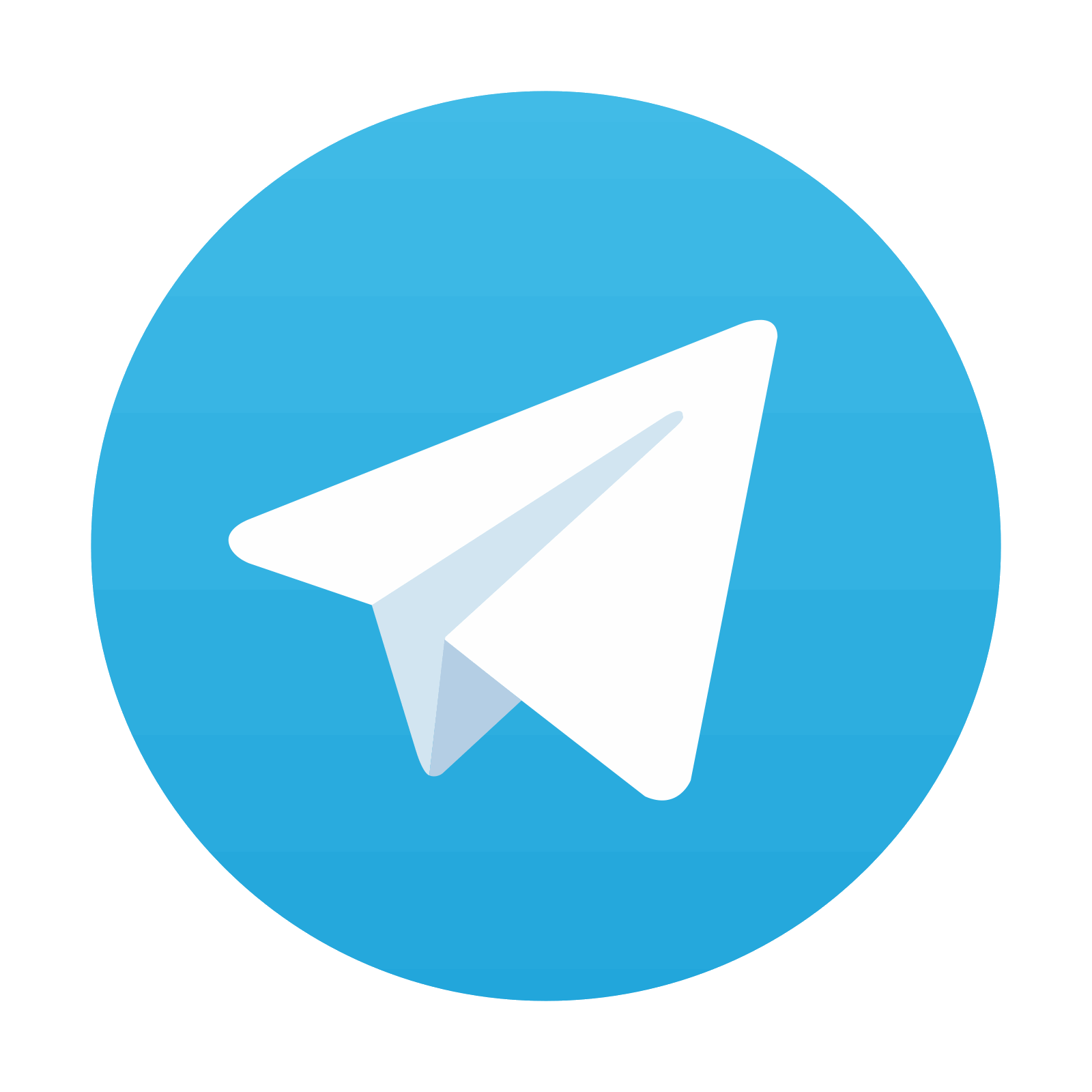
Stay updated, free articles. Join our Telegram channel
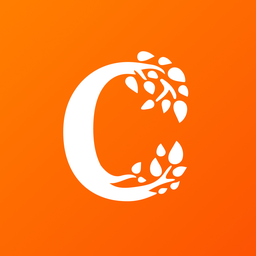
Full access? Get Clinical Tree
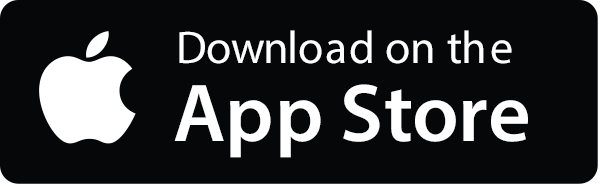
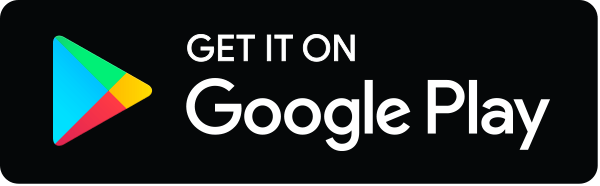