Chapter 5 Biology and Biomechanics of the Tendon–Bone Insertion
Outline
The attachment of a compliant material like tendon to a relatively stiff material like bone is a fundamental engineering challenge.1 As is evident from the study of attachment of engineering materials,2 potentially damaging stress concentrations can be expected to arise at the insertion if the tissue is not tuned to this stiffness mismatch both at the macroscopic level (i.e., the outward splay of the tendon) and the microscopic level (i.e., the gradual stiffening of collagen molecules by mineral crystals).
Structure and Function of the Tendon–bone Insertion
The gradual tailoring, or “functional grading,” of material systems has been described in the engineering materials community as a way to minimize these stress concentrations.3 A central concept to the formation of a robust attachment between tendon and bone is that smooth, monotonic transitions in mechanical properties are necessary for minimizing stresses. The tendon–bone insertion site is a natural functionally graded structure. Notably, this gradation is not maintained at healing insertions, and the surgically repaired tissue is prone to rerupture.4–6 Surgical technique and treatment approaches should therefore seek to minimize stress concentrations and thereby protect the repair site from rupture.
Morphology of the Tendon Enthesis (tendon–bone Insertion Site)
Tendons insert into bone across broad fibrous transitions or across short fibrocartilaginous transitions.7 Fibrous transitions are characterized by insertion sites with large footprints (effectively distributing the forces over large areas and reducing stresses) and by perforating mineral fibers (“Sharpey’s fibers”). Examples of these attachments include the tibial insertion of the medial collateral ligament and the deltoid tendon insertion into the humeral head. Examples of the more common fibrocartilaginous insertions include the rotator cuff tendon insertions and the Achilles tendon insertion. The structure, function, development, and healing of fibrocartilaginous tendon–bone insertions will be the focus of this chapter. These insertions are characteristically short and occur across distinct fibrocartilage regions classically categorized into four zones (Figure 5-1).7 The first zone consists of tendon; this region is populated by fibroblasts and has properties similar to those found at the tendon mid-substance. It is composed primarily of well aligned type I collagen fibers.8 The second zone consists of fibrocartilage; this region is populated by fibrochondrocytes and is composed of types I, II, and III collagen and the proteoglycan aggrecan.8–11 The third zone is also populated by fibrochondrocytes and is composed of mineralized fibrocartilage. Here, the predominant collagen is type II, and there are significant amounts of type X collagen as well as aggrecan.8–11 The fourth zone consists of bone proper; this region is populated by osteoblasts and osteoclasts and is composed predominantly of mineralized type I collagen. While the insertion site is typically categorized into these four zones, changes in the tissue are gradual and continuous, minimizing stress concentrations between the tendon and the bone. The complexity of the tendon–bone insertion, however, results in a difficult challenge for effective healing.
Gradations in Biomechanical, Compositional, and Structural Properties Along the Tendon–Bone Insertion Site
The tendon–bone insertion contains gradients in biomechanical, compositional, and structural properties. Between the unmineralized and mineralized fibrocartilage of the enthesis is a narrow zone that darkens markedly during tissue staining. This “line” was traditionally thought to represent a mineralization front, or, mechanically, a boundary between soft and hard tissue. Studies examining the supraspinatus tendon–bone insertions of rats, however, have demonstrated gradations in properties along the enthesis rather than an abrupt change from mineralized to unmineralized tissue. There was a variation in gene expression along the length of the insertion; tendon-specific genes were localized to the tendon proper, bone-specific genes were localized to the bone proper and the mineralized portions of the insertion, and cartilage specific genes were localized to the insertion.12 Collagen fibers were less oriented at the insertion compared to the tendon.12 Mineral content increased linearly from tendon to bone.13 Biomechanically, both the elastic and viscous behaviors of the tissue varied from tendon to bone.12 The tensile properties of the tendon, for example, were stiffer than those of the insertion. Structure-function relationships were also examined at the anterior cruciate ligament–bone insertion.14 Microcompression was performed to determine the compressive properties and energy dispersive x-ray analysis was performed to determine mineral content along the ligament enthesis. The calcified regions of the insertion exhibited significantly greater compressive moduli than the noncalcified regions. Based on these results, it is apparent that there is a gradation along the tendon–bone insertion site with regard to collagen structure, extracellular matrix composition, mineral content, and biomechanical properties.
Composition and Microstructure of the Insertion Site Is Optimized to Minimize Stress Concentrations
Mechanical models of the insertion demonstrate that the orientation of the collagen fibers and their level of mineralization are optimized to minimize stress and strain concentrations. In one study, a finite element model of the insertion was used to determine the role of collagen orientation on stress and strain distributions.15 The idealized model showed that the microstructure serves to reduce stress concentrations and material mass and to shield the insertion’s outward splay from the highest stresses. Similarly, a model of the medial collateral ligament femoral insertion demonstrated that the direction of the principal tensile stresses coincides with the direction of the collagen fibers.16 A correlation was found between cell shape and mechanical stresses, suggesting a direct relationship between the stress field and cell activity. The effect of mineral content on load transfer was modeled at the insertion in a separate study.17 It was demonstrated that mineral content and collagen fiber orientation combine to give the tendon–bone transition a unique grading in mechanical properties, supporting the idea for a functionally graded, continuous tissue transition from tendon to bone. An increase in mineral on collagen fibers provided significant stiffening of the fibers. The orientation of collagen fibers was the second major determinant of tissue stiffness. The combination of these two factors resulted in a variation of stiffness over the length of the tendon–bone insertion similar to what has been described from experimental testing.12,18 These biomechanical models describe how tendon–bone attachment is achieved in nature through a functionally graded material composition.
Development of the Tendon–bone Insertion Site
Studying the development of the tendon enthesis may provide insights into the biological processes necessary to form this complex anatomical site. These insights are critical for the success of biological treatments or tissue engineering solutions for tendon–bone repair. Animal models indicate that the enthesis develops into a functionally graded tissue postnatally,19–22 allowing a unique opportunity to study and perturb its formation.
Role of Biological Factors
Several factors are important to the development of the tendon–bone insertion site. Scleraxis is a transcription factor expressed both in tenocyte progenitors and in mature tendon cells and has been associated with tendon and insertion site formation.23 SRY (sex determining region Y)-box 9 (SOX-9) is associated with chondrogenesis and has been localized in proliferating chondrocytes.24 Parathyroid hormone–related protein (PTHrP) and Indian hedgehog (IHH) drive chondrocyte proliferation, but also form a negative feedback loop, maintaining a population of proliferative cells at the growth plate, available for further growth and differentiation.25 These factors are seen in the growth plate of developing bones and likely play a similar role at the formation of the tendon enthesis, as many of the same cell types and maturation processes are seen at both anatomical locations.26
The developing growth plate contains cells that are divided into distinct zones based on morphology and mineral content.27 The zones are the reserve zone, the proliferative zone, and the hypertrophic zone. The small cells in the reserve zone proliferate and organize into columns in the proliferative zone, and finally become hypertrophic chondrocytes. It is at the leading edge of the hypertrophic zone that calcium is deposited in the zone of provisional calcification leading to mature bone. Similar chondrocytes are seen at the developing insertion site; these cells eventually mineralize to form a tendon enthesis with a gradation in mineral.
The tendon–bone insertion site develops in the early postnatal period.19–22 Mouse studies have elucidated the time course of development of the tendon enthesis using in situ hybridization.20,21 Collagens were localized along the developing insertion. Collagen I was examined due to its prevalence in both tendon and bone. Collagen II was examined due to its association with chondrocytes, and hence the fibrocartilage region of the insertion. Last, collagen X was examined due to its reported expression by hypertrophic chondrocytes of the growth plate. Rotator cuff tendon precursors were juxtaposed to the humeral head at 15.5 days postconception. A transition zone between the developing tendon and bone was not apparent until 7 days after birth. Prior to 14 days postnatally, collagen I was consistently expressed on the tendon side of the insertion while collagen II was consistently expressed on the humeral side, mirroring each other during these early time periods. Collagen X was expressed at 14 days in association with hypertrophic chondrocytes. As the humeral head matured and mineralized, it began to express collagen I, the main collagen type in mature bone. A mature fibrocartilaginous insertion site was formed by 21 days postnatally. This corresponded to the completion of mineralization of the cartilaginous humeral head. Similar results were seen at the developing Achilles tendon insertion of the rat21 and human.28
PTHrP has been localized to the insertion sites of both tendons and ligaments.26 In the growth plate, it is seen in proliferating chondrocytes, and is known to be part of a negative feedback loop, preventing their maturation into hypertrophic chondrocytes, which then mineralize to form bone. Therefore, it plays an important role not only in maintaining a population of cells available for growth but also for preventing inappropriate mineralization. It likely plays an analogous role in maintaining a barrier between mineralized and unmineralized tissues at the tendon to bone insertion site. PTHrP works in concert with other factors. IHH is secreted by proliferative chondrocytes before they become hypertrophic chondrocytes. It binds to the cell membrane receptor Patched 1 leading to the accumulation of a transmembrane protein smoothened, which leads to the production of PTHrP. These factors are all localized to the developing tendon insertion site at 14 to 21 days, correlating to the onset of mineralization of the humeral head. These factors are also highly mechanosensitive.29 Specifically, their expression is decreased when load is removed.
SOX-9 and scleraxis are important factors in chondrogenesis and tenogenesis, respectively.30 SOX-9 is localized to proliferating chondrocytes, but not in hypertrophic chondrocytes, and may play some role in insertion site development. Serving as a marker for many connective tissues, including ligaments, tendons, and joint capsules, scleraxis has been localized at the developing insertion site and is required for tenogenesis. Scleraxis likewise has been shown to be highly sensitive to the mechanical environment, specifically, upregulated in stem cells exposed to tensile forces.31
Role of Mechanical Factors
A series of experiments using mice were performed to elucidate the effects of the mechanical environment on the formation of the tendon–bone insertion site.19 Botulinum toxin A was injected to the shoulders of mice within 24 hours of birth, simulating a neonatal brachial plexus injury and removing muscle load from the humeral head. Saline injections in the contralateral shoulders and a separate group of healthy mice were used as controls. Many effects were observed due to paralysis in bony development, soft tissue contracture, muscle mass, and muscle force generation. Dramatic changes were seen at the developing tendon–bone insertion site due to unloading.
The sensitivity of bone to its mechanical environment was highlighted in this series of experiments. Removal of load significantly decreases amounts of bone mineral in the humeral heads of the paralyzed mice (Figure 5-2)
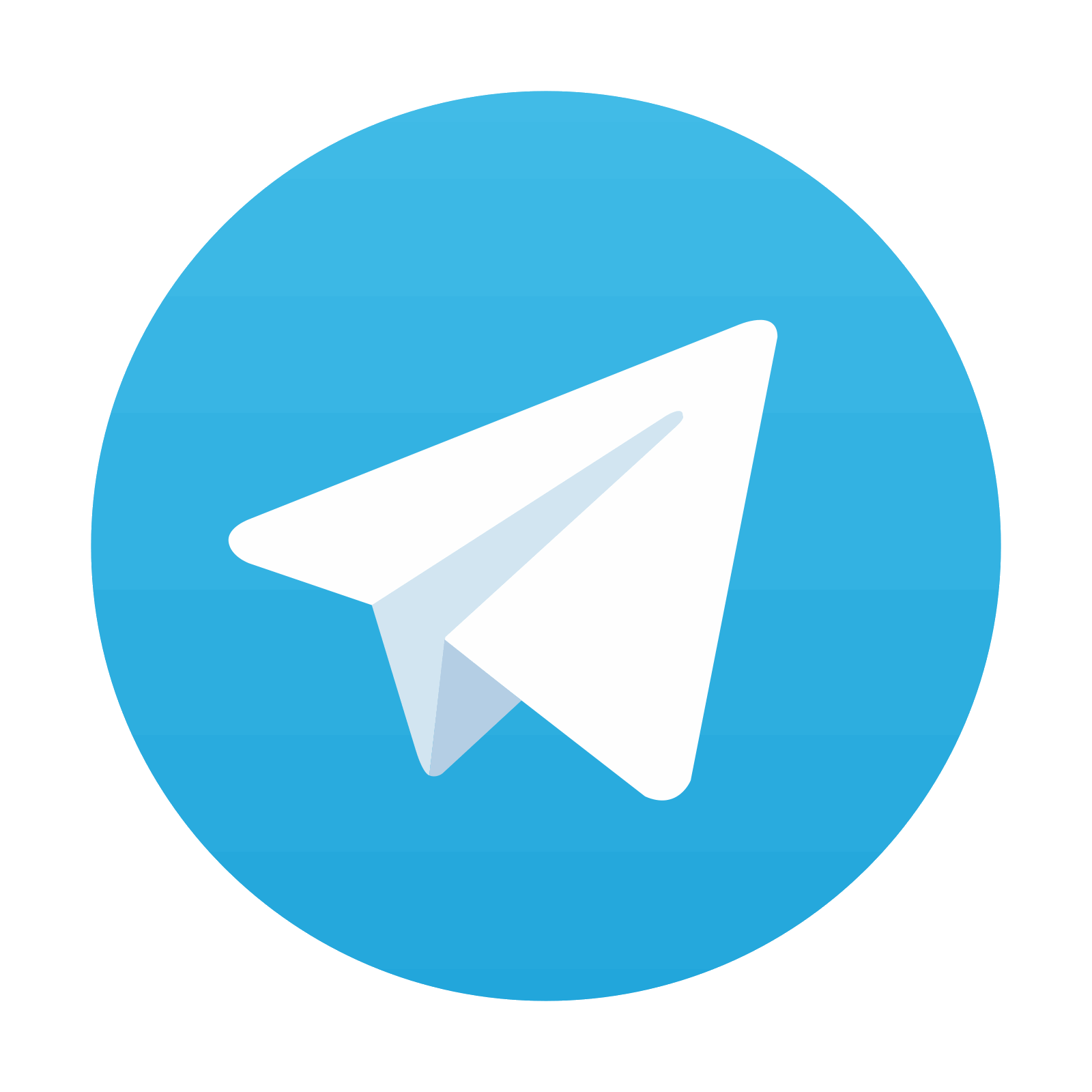
Stay updated, free articles. Join our Telegram channel
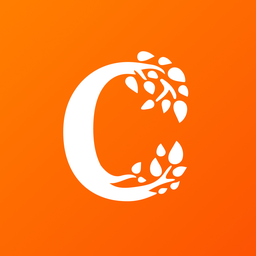
Full access? Get Clinical Tree
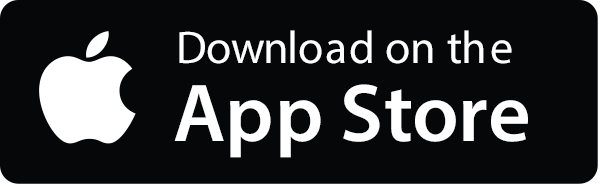
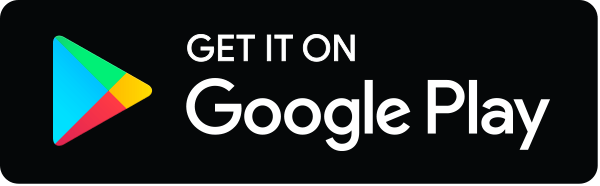