Abstract
Human disease can arise from genetic changes ranging from mutations in single nucleotides to alterations affecting entire chromosomes. Genetic skin diseases include Mendelian (monogenic) disorders caused by defects in one gene as well as polygenic disorders due to defects in more than one gene; complex (multifactorial) disorders result from the interaction of environmental and genetic factors. Tremendous progress has been made in determining the genetic basis of human diseases. Functional and positional cloning as well as positional candidate approaches have been utilized to identify pathogenic mutations for Mendelian disorders, while genome-wide association studies (GWAS) and functional genomics have more recently been employed to investigate complex disorders. The advent of massively parallel sequencing technology has also enabled identification of novel pathogenic variants. A basic understanding of genetics and the methods currently used to study genetic diseases is essential for the incorporation of research advances into patient care.
Keywords
genodermatoses, gene, mutation, monogenic, polygenic, Mendelian disorder, autosomal dominant, autosomal recessive, X-linked dominant, X-linked recessive, mosaicism, chromosomal disorders, incomplete penetrance, variable expression, de novo mutations, pseudodominant inheritance, genomic imprinting, mitochondrial inheritance, functional cloning, positional cloning, linkage analysis, single nucleotide polymorphism (SNP), comparative genomic hybridization (CGH), copy number variation, loss of heterozygosity, genome-wide association studies (GWAS), massively parallel sequencing, whole-exome sequencing, whole-genome sequencing, functional genomics
Introduction
The completion of the human genome sequence, with an estimated size of 3.2 gigabases (Gb) and containing ~20 000–25 000 genes , represents a powerful tool in medicine that facilitates the identification of disease-associated genes. Detailed information on human sequence variation and advanced technologies for its detection have broadened our knowledge of the genetic basis of human disease. The pathogenesis of many disorders also involves epigenetics, which represent heritable changes in phenotype and/or gene expression that do not result from changes in the DNA sequence.
Rapid advances in the development of molecular biology techniques (see Ch. 3 ), together with information-based tools derived from the sequencing of the human genome, provides the physician with direct access to clinically relevant genetic information. Consequently, it is important for physicians in general, and dermatologists in particular, to understand basic concepts of genetics ( Table 54.1 ). Familiarity with new technology and approaches to identifying disease-causing genes is essential in order to appropriately utilize and interpret the results of genetic studies in the patient care setting. The wealth of new information emphasizes the need to bridge the gap between the bench and the bedside, bringing together efforts from both the clinical and research arms of dermatology.
BASIC CONCEPTS IN GENETICS | |
---|---|
Nuclear DNA |
|
Chromosome |
|
Karyotype |
|
Haploid cell |
|
Diploid cell |
|
Centromere |
|
Telomere |
|
Meiosis |
|
Mitosis |
|
Locus |
|
Gene |
|
Allele |
|
Polymorphic locus |
|
Single nucleotide polymorphism (SNP) |
|
Mutation |
|
Genotype |
|
Phenotype |
|
Access to patients with genetic disorders and accurate clinical descriptions are essential for investigations aimed at the identification of disease-causing genes. Combined with functional studies and translational approaches, these findings can in turn provide benefits to affected individuals. Such insights have importance in genetic counseling and prenatal diagnosis, and they can also contribute to the identification of at-risk individuals/families, prediction of disease course and possible complications, determination of response to therapeutic interventions, and even the development of targeted treatments.
Since the early 1980s, the molecular bases of numerous cutaneous genetic disorders have been elucidated, including multiple forms of epidermolysis bullosa (EB; see Ch. 32 ), ectodermal dysplasia (ED; see Ch. 63 ), ichthyosis (see Ch. 57 ), and hypotrichosis (see Chs 68 & 69). The gene-identification strategies employed for these relatively uncommon Mendelian (single-gene) disorders have provided a foundation for the study of common complex disorders such as atopic dermatitis, psoriasis, and alopecia areata. Advances in mapping and detecting human sequence variation have led to more sophisticated approaches, including genome-wide association studies (GWAS) and whole-exome sequencing. Functional genomic studies, e.g. comparison of the transcriptome (expressed genes) in samples from patients and unaffected individuals, have also helped to identify candidate genes for further investigation. The clinician plays a key role in enabling such research, which requires a well-characterized collection of patient samples.
Genetic Diseases
- ▪
Accurate information about phenotype and family history is essential in identifying the pattern of inheritance of a genetic disease
- ▪
Genetic diseases include Mendelian (monogenic) disorders caused by defects in one gene as well as polygenic disorders due to defects in more than one gene; complex (multifactorial) disorders result from the interaction of environmental and genetic factors
- ▪
The major Mendelian patterns of inheritance are autosomal recessive (e.g. oculocutaneous albinism), autosomal dominant (e.g. Darier disease), X-linked recessive (e.g. hypohidrotic ectodermal dysplasia), and X-linked dominant (e.g. incontinentia pigmenti)
- ▪
Factors modifying the basic Mendelian pattern of inheritance include incomplete penetrance, age-dependent penetrance, variable expression, de novo mutations, pseudodominant inheritance, genomic imprinting, and mitochondrial inheritance
- ▪
Chromosomal anomalies can consist of abnormalities in the number (e.g. polyploidy, aneuploidy) or structure (e.g. translocations, inversions) of the chromosomes
- ▪
Heterogeneity at the locus or allelic level can make identification of a gene defect challenging
Mendelian (monogenic) diseases are caused by defects in one gene, while polygenic diseases result from defects in more than one gene and complex (multifactorial) diseases result from the interaction of environmental and genetic factors. In addition to mutations within particular genes, abnormalities in the structure, number, or parental contribution of chromosomes can cause disease.
In the following sections, we provide an overview of the different types of genetic diseases.
Mendelian Patterns of Inheritance
A key initial step in the assessment of the risk for a particular inherited disease is determination of its mode of inheritance. For this purpose, it is essential to collect accurate information on the clinical phenotype and to examine as many family members as possible to classify their status – affected or unaffected. The family is usually ascertained through one family member, the proband , around whom the pedigree is built with information on the phenotypes and relationships between different individuals.
Even when a single-gene defect is enough to cause a disease phenotype (monogenic disorders), the clinical expression can be affected by variable expression and even incomplete penetrance (see below). Genes are expressed on a particular genetic background, which is different in every individual, and genes and their products interact with other genes and proteins. One can therefore expect that monogenic disorders will show some degree of variability.
Allelic heterogeneity refers to mildly or even considerably different clinical entities due to different mutations in the same gene ( Table 54.2 ). In contrast, locus (genetic) heterogeneity refers to mutations in different genes that result in the same phenotype ( Table 54.3 ). Of note, there are some diseases, such as EB simplex, that display both allelic and locus heterogeneity. Thus, diagnosis based purely on either clinical signs/symptoms or the results of genetic testing can lead to misclassification, underscoring the importance of integrating clinical and molecular findings.
EXAMPLES OF ALLELIC HETEROGENEITY IN GENODERMATOSES | |
---|---|
Mutated gene | Phenotypes |
GJB3 | Erythrokeratodermia variabilis AR and AD non-syndromic hearing impairment AD peripheral sensory neuropathy and hearing impairment |
Desmoplakin ( DSP ) | Striate palmoplantar keratoderma Dilated cardiomyopathy with woolly hair and keratoderma (Carvajal syndrome) Severe dermatitis, multiple allergies, and metabolic wasting (SAM) syndrome Skin fragility–woolly hair syndrome Acantholytic EBS Arrhythmogenic right ventricular cardiomyopathy |
ABCA12 | Harlequin ichthyosis Lamellar ichthyosis |
Plectin ( PLEC ) | EBS and limb-girdle muscular dystrophy EBS of the Ogna type EBS with pyloric atresia |
ATP7A | Menkes disease Occipital horn syndrome |
EXAMPLES OF GENODERMATOSES WITH GENETIC/LOCUS HETEROGENEITY | |
---|---|
Phenotype | Gene |
Striate palmoplantar keratoderma | DSG1 DSP KRT1 |
Epidermolytic ichthyosis | KRT1 KRT10 |
Epidermolysis bullosa simplex (localized and generalized variants) | KRT5 KRT14 |
Hermansky–Pudlak syndrome (HPS) | HPS1, HPS3, HPS4, HPS5, HPS6 AP3B1, AP3D1 DTNBP1, BLOC1S3, BLOC1S6 |
Tuberous sclerosis complex (TSC) | TSC1 TSC2 |
Hypohidrotic ectodermal dysplasia * | EDA (ectodysplasin A) EDAR (ectodysplasin A receptor) EDARADD (EDAR-associated death domain) |
* Mutations in different genes result in X-linked recessive (EDA), autosomal recessive (EDAR, EDARADD), and autosomal dominant (EDAR, EDARADD) forms of the disorder.
Once a phenotype and its range of clinical findings are defined, the trait can be followed within a pedigree. The transmission of the trait in the pedigree defines the pattern of inheritance of the disease. The genes responsible for monogenic traits can be located either on autosomes, resulting in an autosomal pattern of inheritance, or on the X chromosome in the case of an X-linked pattern of inheritance. Irrespective of the gene location, when a mutation in one of two alleles is sufficient to produce the phenotype, the trait is considered dominant . In contrast, when both alleles of a gene (or one allele in hemizygotes) must be altered in order to produce the phenotype, the condition is considered recessive . Hence, Mendelian inheritance patterns include autosomal dominant, autosomal recessive, X-linked dominant, and X-linked recessive ( Table 54.4 ). There are also Y-linked genes that primarily affect male fertility , although their relevance in more general diseases is possible.
PATTERNS OF INHERITANCE | |||||
---|---|---|---|---|---|
Pattern | Parents affected | Gender affected | Transmission | Recurrence risk | Risk factors |
Autosomal recessive | No (carriers) | Both equally | Disease seen in siblings of proband, not in parents or offspring Usually only in one generation | 1 in 4 | Consanguinity, isolated population (e.g. geographically, linguistically) |
Autosomal dominant | Yes * | Both equally | Disease seen in successive generations | 1 in 2 | De novo mutations |
X-linked recessive | Mother a “carrier” * | Males have the “complete” disease Female “carriers” may have mild manifestations (e.g. in a mosaic pattern) ** | No male-to-male transmission (but all daughters of an affected male are “carriers”) | 1 in 2 male children born to a female “carrier” will be affected (and 1 in 2 of her female children will be carriers) | De novo mutations |
X-linked dominant | Yes * | Predominantly females if lethal in males during embryonic development; otherwise milder in females (often with a mosaic pattern of skin lesions) and more severe in males | Affected males have: (1) no affected sons; and (2) all daughters affected No male-to-male transmission | 1 in 2 children born to affected female; may spontaneously abort male fetuses if “male-lethal” condition | De novo mutations |
* Unless the proband has a de novo mutation and is therefore the first generation affected.
** Does not represent a “pure” X-linked recessive disorder if there are manifestations in female “carriers”.
This chapter focuses on genodermatoses, but the explanations of Mendelian and complex traits also apply to extracutaneous diseases.
Autosomal Dominant Inheritance
In a pedigree of an autosomal dominant disease, each affected person other than the first affected family member, who has a de novo mutation, has an affected parent. Both sexes are equally affected and can transmit the trait, and there is vertical transmission from generation to generation ( Fig. 54.1A ) . Since a single mutant allele is enough to produce the phenotype, each offspring of an affected individual has a 50% risk of inheriting the mutant allele and therefore the disorder. Examples of autosomal dominant genodermatoses include EB simplex, Darier and Hailey–Hailey diseases, and nail–patella syndrome.

A patient with an autosomal dominant disease is usually heterozygous for the disease-causing mutation. Homozygosity for autosomal dominant traits occasionally occurs, and this usually results in a more severe phenotype. For example, a mutation in the keratin 14 gene ( KRT14 ) may give rise to localized EB simplex when heterozygous but generalized EB simplex when homozygous . Exceptions to the expected autosomal dominant inheritance pattern may also be due to incomplete penetrance or variable expression (see below).
Dominant mutations can give rise to a phenotype by: (1) haploinsufficiency, in which the protein produced from one wild-type (non-mutant) allele is not sufficient to sustain normal function; or (2) a dominant negative effect , where the mutated proteins physically interfere with wild-type proteins and prevent them from functioning properly. The latter mechanism often occurs when the mutated protein forms dimers or polymers; examples include keratins (e.g. KRT14 in EB simplex) and tyrosine kinase receptors (e.g. KIT in piebaldism).
Autosomal Recessive Inheritance
In a pedigree of an autosomal recessive disease, an affected individual has clinically unaffected parents, both sexes are equally affected, and there is no transmission from generation to generation ( Fig. 54.1B ). Both alleles of a given gene must be mutated for the disease to develop. When both parents are heterozygous carriers of a mutant allele, the chance of inheriting the disorder is 25% for each of their offspring. Oculocutaneous albinism type 1 and atrichia with papular lesions are examples of autosomal recessive genodermatoses.
There is often an increased frequency of consanguinity (sharing a common ancestor) in families with recessive conditions. Consanguineous matings (inbreeding) increase the probability that a mutated allele present in the common ancestor will become homozygous in the offspring (homozygosity by descent); these individuals are typically also homozygous for the region surrounding this mutated gene. When more than one affected family member with an autosomal recessive disease is present in a single pedigree, they are usually part of the same generation. However, the presence of consanguinity can also explain some pedigrees in which an autosomal recessive trait is observed in more than one generation. For example, matings between unaffected carriers and affected patients can give rise to affected family members in two consecutive generations (pseudo-dominant inheritance; see below).
Patients with an autosomal recessive disease have two mutant alleles (biallelic mutations), while both of their parents are typically heterozygous carriers of one mutant allele as well as having one wild-type allele. If both parents carry the same mutation, their affected offspring will be homozygous for the disease-causing mutation; this is the usual mechanism in consanguineous families. However, the parents may carry two different mutations in the same gene, and their affected offspring will be compound heterozygous .
X-Linked Recessive Inheritance
Patients with an X-linked recessive disease are typically male, and they classically have unaffected parents. However, in several X-linked “recessive” disorders, patients’ mothers have some clinical evidence of the condition due to functional mosaicism (see Ch. 62 ). These mosaic manifestations in female “carriers” blur the distinction between recessive and dominant forms of X-linked inheritance, thereby removing such conditions from the “pure” X-linked recessive category. With variable skewing of X-inactivation (see below), the spectrum of disease in female “carriers” can range from absent to severe.
There is no male-to-male transmission, since an affected male will transmit the Y chromosome and not the mutant X chromosome to his son, and the complete (non-mosaic) trait does not appear in successive generations ( Fig. 54.1C ). However, one rare exception is the offspring of an affected male and a female carrier.
The inheritance risk for the offspring depends on the parent transmitting the disease allele. An affected father will transmit the mutated allele (but not the complete disease) to all his daughters, but to none of his sons. Thus, his daughters become obligate carriers and will transmit the mutated allele to 50% of their children. As a result, the sons and daughters of a carrier mother will have a 50% chance of being affected or carriers, respectively.
Examples of recessive X-linked diseases in dermatology are X-linked recessive ichthyosis (steroid sulfatase deficiency; see Ch. 57 ), hypohidrotic ectodermal dysplasia, and congenital generalized hypertrichosis.
X-Linked Dominant Inheritance
In a pedigree of an X-linked dominant disease, affected individuals have an affected parent, with the exception of the first affected family member. Unless male-lethal, these disorders occur in both males and females. However, because females are heterozygous rather than hemizygous, they generally have a milder and/or mosaic phenotype (see below). There is no male-to-male transmission, and the trait appears in successive generations ( Fig. 54.1D ). The risk of the offspring inheriting the disease depends on the transmitting parent. An affected father will transmit the trait to all his daughters, but to none of his sons. An affected mother, on the other hand, will transmit the trait to 50% of her children, regardless of the sex of the offspring.
Some X-linked dominant diseases are lethal in males during early intrauterine development. In this situation, the disorder is seen almost exclusively in females, and when these women become pregnant they may have an increased frequency of spontaneous abortions. Occasional male patients can be explained by mosaicism, either functional in the setting of Klinefelter syndrome (47, XXY karyotype) or genomic due to mosaicism secondary to a de novo half-chromatid or postzygotic mutation (see Ch. 62 ).
Because female embryos undergo lyonization, i.e. the random epigenetic inactivation of one of the two X chromosomes in each cell, female patients with X-linked dominant skin diseases typically have a mosaic pattern of cutaneous lesions (see Ch. 62 ). Non-random patterns of X-inactivation in these patients may reflect preferential survival of cells expressing the normal X chromosome. Examples of X-linked dominant, male-lethal diseases in which girls present with a mosaic pattern of skin findings are focal dermal hypoplasia (Goltz syndrome) and incontinentia pigmenti .
Exceptions to Basic Mendelian Patterns of Heritance
Although the genotype at a single locus is responsible for the development of a Mendelian disease, other factors can affect the phenotypic manifestations and pattern of inheritance. Exceptions to basic Mendelian inheritance patterns are presented in Table 54.5 and Fig. 54.2 .
EXCEPTIONS TO BASIC MENDELIAN INHERITANCE PATTERNS | |||
---|---|---|---|
Type of exception | Impact on phenotype and inheritance | Examples in dermatology | Impact on genetic counseling |
Variable expression |
| Broad ranges of severity in Darier disease and neurofibromatosis type 1 |
|
Incomplete/reduced penetrance |
| Hereditary leiomyomatosis and renal cell cancer syndrome |
|
Age-dependent penetrance |
| Late onset in Darier and Hailey–Hailey diseases |
|
X-inactivation (lyonization; see Ch. 62 ) * |
| Skewed X-inactivation favoring the wild-type allele in the skin of girls with incontinentia pigmenti and the hematopoietic cells of female carriers of X-L dyskeratosis congenita |
|
De novo mutations |
| De novo mutations account for ~30–50% of patients with NF1 and >60% of those with tuberous sclerosis complex |
|
Genomic mosaicism: somatic and gonadal (see Ch. 62 ) |
| Transmission via gonadal mosaicism has been documented in NF1, dominant dystrophic EB, and epidermolytic ichthyosis (± an epidermolytic epidermal nevus reflecting somatic mosaicism in the parent) |
|
Type 1 and 2 mosaicism/ segmental manifestations in AD disorders (see Ch. 62 ) |
| Type 1: epidermal nevi, linear Darier disease Type 2: linear porokeratosis superimposed on disseminated superficial actinic porokeratosis, segmental Hailey–Hailey disease in the setting of milder classic symmetric disease |
|
Loss of heterozygosity in AD tumor predisposition syndromes |
| Skin tumors in hereditary leiomyomatosis and renal cell cancer and basal cell nevus (Gorlin) syndrome |
|
Revertant mosaicism ** (see Ch. 62 ) |
| Several types of EB, ichthyosis with confetti |
|
Consanguineous mating (inbreeding) |
| A founder effect due to ancestors (Dutch settlers) carrying a recessive mutation explains the high incidence of porphyria variegate in Caucasian South Africans |
|
Quasidominant (pseudodominant) inheritance |
| Consanguineous families with atrichia with papular lesions or pseudoxanthoma elasticum |
|
Mitochondrial inheritance |
| Mutation in the mitochondrial serine tRNA gene underlying palmoplantar keratoderma with or without associated sensorineural deafness |
|
Phenocopy |
| A clinical presentation similar to porphyria cutanea tarda (PCT; an AD disorder) can result from exposure to certain hydrocarbons and pesticides |
|
Imprinting and uniparental disomy (UPD) |
| Prader–Willi and Angelman syndromes (see Fig. 54.3B ); uniparental isodisomy involving a recessive mutant allele has been reported in junctional EB, recessive dystrophic EB, and Harlequin ichthyosis |
|
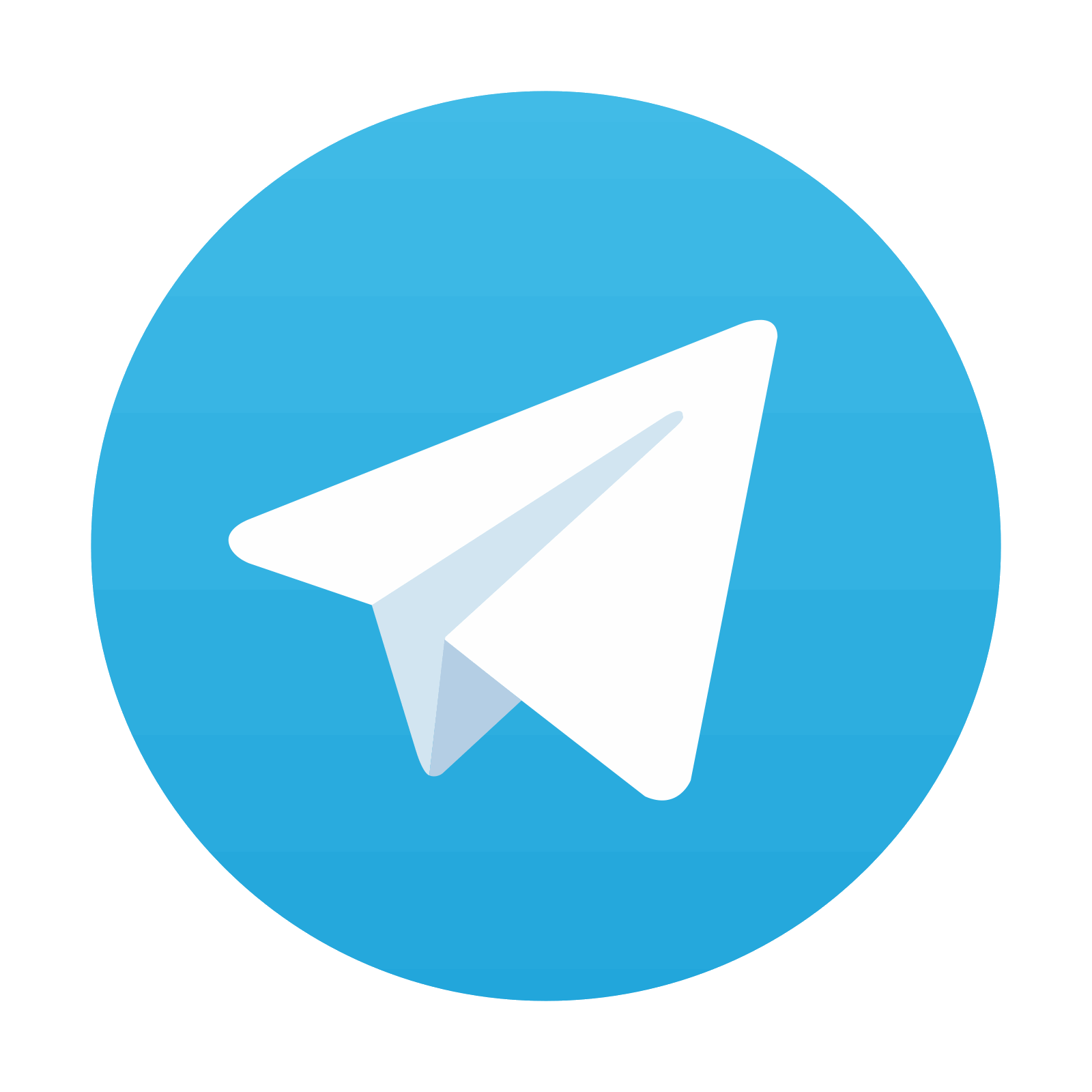
Stay updated, free articles. Join our Telegram channel
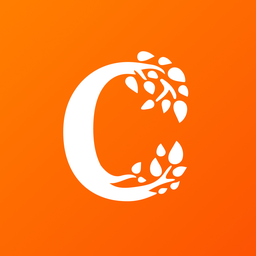
Full access? Get Clinical Tree
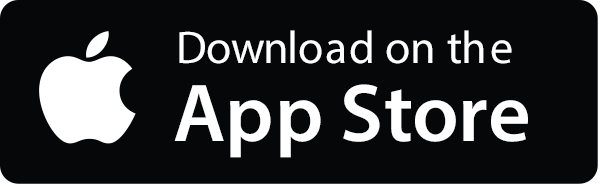
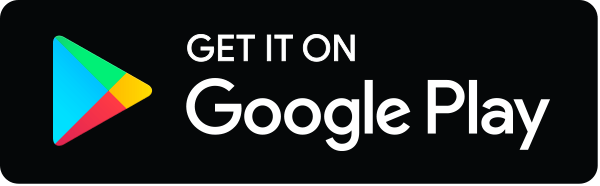
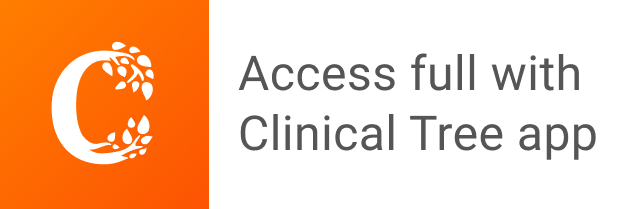