60 Basic Principles of Craniofacial Bone Healing and Repair
Introduction
This chapter reviews basic concepts and principles of bone formation and repair important for facial plastic surgeons. Surgery of the craniofacial skeleton is an integral part of facial plastic surgery and thus a critical knowledge of fundamental bone biology and applied biotechnology including cellular, genetic, and molecular biology of bone formation and repair has become a requirement for successful clinical care. From this expanding knowledge, increasingly sophisticated surgical techniques of bone repair and fixation have permitted the development of more effective treatments and resultant unprecedented successful patient outcomes.
Bone Formation and Structure
Bone tissue is a specialized composite of extracellular matrix proteins that is mineralized under the surveillance of associated cells, which maintain the structural integrity of the bone while responding to the metabolic requirements of the organism.
Formation
During embryologic development there are two primary processes of bone formation. Intramembranous bone formation occurs independently of a preexistent model or structure; endochondral bone formation occurs via replacement of a cartilaginous structure or model/template.
In membranous bone formation, the ossification process takes place by direct mineral deposition into the organic matrix of mesenchymal tissues. The mesenchymal cells receive signals to induce differentiation to an osteoblastic lineage. Within the craniofacial skeleton, this is the major process observed. The frontal and parietal bones, nasal bone, maxilla, zygoma, and mandible are all of membranous origin.
In endochondral bone formation, mesenchymal cells differentiate to form a cartilage template. The cartilaginous structure proceeds through a hypertrophic state to become mineralized and ultimately replaced by differentiated bone cells. The removal and replacement of the mineralized cartilage is accomplished by the invasion of the template structure by osteoclast (large multinucleated) cells and blood vessels. Following removal of the mineral matrix, osteogenic precursor cells repopulate the template structure and form bone. This mechanism of bone formation is the basis of the axial skeleton; in the craniofacial region, endochondral origin is observed in the nasal septum and portions of the nasal bone complex, the occipital bone, skull base, and mandibular condyle.
Appositional growth in all bones (of either embryologic origin) proceeds via membranous bone formation. Due to ongoing metabolic modeling and remodeling processes, no remnants of calcified cartilage are detected. The role that bone origin might play in healing and repair versus the impact of local regulatory factors has not been fully established. Many new insights regarding the molecular biology of bone formation are now being revealed, such as the interplay between developmental gene control for limb development (e.g., hox/HOM gene network) and local regulation (e.g., parathyroid hormone–related protein). Platelet-derived growth factor (PDGF) plays a role in inducing proliferation of undifferentiated mesenchymal cells. PDGF-AA and PDGF-BB have been shown to enhance proliferation of multiple types of bone cells, including both osteoblast and osteoclast lineages. The stimulatory effect of PDGF on bone proliferation appears dependent on the donor age and stage of cellular differentiation in that responsiveness to PDGF is decreased in more differentiated cells. Insulinlike growth factors are partly responsible for the general growth and maintenance of the body skeleton, with high levels of insulinlike growth factor-1 rapidly activating bone turnover with an increase in serum osteocalcin and carboxyterminal propeptide of collagen I as a marker of bone formation as well as an increased urinary ratio of calcium/creatinine and deoxypyridinoline excretion (a marker of increased bone resorption). Transforming growth factor β (TGF-β) has a biphasic effect, with enhancement of bone formation at low concentrations and suppression of proliferation and osteoblastic differentiation at high concentrations. Bone morphogenic proteins (BMPs), especially BMP2, BMP4, and BMP7, appear to be the most important and potent growth factors in terms of osteogenesis. Although beyond the scope of this chapter, further elucidation of the biology of bone formation will have an impact on clinical bone repair in the future.
Gross Structure
Grossly, bone can be descriptively classified as either cortical or cancellous. These terms refer only to the structural architecture, not cellular origin or composition. Cortical (or compact) bone is immediately beneath the periosteum, adjacent to the endosteum, and these surfaces are lined with envelopes providing vascularity and osteoprogenitor cells for repair. The envelopes form a system of lacunae and canaliculi around the mineralized matrix for the transport of metabolites; this limits the outer diameter of the osteon (or basic bone unit) to ∼ 100 µm of maximum diffusion and 200 µm of trabecular plate width. Within the cancellous bone, trabecular architecture is often described. The size, volume density, and dimension are variable and depend on the age, site, and loading of the bone. The transitions between the compact and cancellous bone are an area of flux; thus, osteoclast cells may erode cavities inside of compact bone, allowing for cancellous bone to form. Conversely, osteoblasts may fill spaces within the trabeculae of cancellous bone to form new compact bone.
On the next level of microscopic exam, bone is usually classified as woven or lamellar, although some authors take the classification still further. Woven bone is characterized by its random collagen fibril orientation. Woven bone forms rapidly on the order of 3 to 5 µm/day in humans and can cover a relatively large physical territory, with resultant diminished biomechanical properties. Lamellar bone is characterized by elaborate orientation, and therefore its formation is more demanding and timeconsuming. Lamellar bone requires a preformed solid scaffold for its deposition; there is strict parallelism of the lamellae to the underlying surface. Any irregularities of the surface are usually addressed by woven bone prior to lamellar bone deposition. The linear appositional bone formation of lamellar bone is ∼ 1 to 2 µm/day.
Remodeling of bone is a concerted and coupled action of bone resorption and formation that takes place on the surface and interior of compact bone. During remodeling, osteoclasts drill or create tunnels. Local signals (growth factors and their regulators) induce osteoblasts to follow and deposit lamellar bone, thereby contracting the tunnel walls around the nutrient capillary, forming secondary osteons, or Haversian systems. Bone remodeling allows for continued renovation and metabolic restoration while preserving functional capacity of the bone structure.
The blood supply to bone in the craniofacial region is abundant. Within the bony structure, the vascular supplies follow the Haversian system and are cross-connected by the Volkmann canals. Peripheral to the capillaries are the canalicular envelopes. These are related spatially to the osteocyte-perfusion exchange as noted earlier, limited to 100 µm. The blood supply to compact bone, especially as its thickness increases, is dependent on long low-pressure connections, thus making it more susceptible to disruption and longer recovery. In contrast, in cancellous bone the vascular supply reaches its anatomical destinations more directly without significant branching. Therefore, all processes of healing and remodeling that would require reestablishment of vascularization occur more rapidly and efficiently. Within the craniofacial bones, the vascular supply is more consistent with the cancellous bone model, with a relatively large surface area to bone volume; as such, these bones are less prone to vascular compromise. The mandible is a mixture of vascular supply types and therefore somewhat more susceptible to compromise. Overall, the plentiful blood supply of the craniofacial skeleton decreases the risk of infective complications in comparison with the extremities and appendicular skeleton.
Biochemical Structure
The noncellular composition of bone matrix is usually divided into organic and nonorganic components. The inorganic bone matrix makes up ∼ 70% of the dry weight of the bone. The major constituent of the inorganic or mineral phase is calcium and phosphate in a unique biologic crystal structure termed hydroxyapatite. Although hydroxyapatite conveys a specific chemical stoichiometry [Ca10(PO4)6(OH)2], various apatitic species of calcium phosphate exist (e.g., amorphous), with hydroxyapatite the most prevalent. The remainder consists of additional ionic species of sodium and magnesium as well as very small concentrations of other inorganic ion species.
The organic matrix of bone is usually divided into collagenous and noncollagenous proteins. Collagen composes 90% of the organic matrix in the form of a bone-specific type I unique in its specific glycosylation and cross-linking. The direct fibril–apatite interaction gives rise to the special biomechanical properties of bone. The remainder of collagen species present in bone are associated with blood vessels or are known to influence fibril diameter (types III, V, XII, and XIII).
The noncollagenous proteins make up the remaining 10% of the organic matrix. They are generally divided into proteoglycans, glycoproteins, Gla-containing proteins, and growth factors. The proteoglycans are composed of glycosaminoglycans covalently linked to core proteins. Examples of proteoglycans are decorin and fibromodulin with associated glycosaminoglycans such as chondroitin and heparin sulfate. These proteoglycans are thought to play a role as binding reservoirs for various growth factors, such as basic fibroblast growth factor and TGF-β.
Glycoproteins are represented by fibronectin, osteonectin, osteopontin, and bone sialoprotein. Glycoproteins share an arginine-glycine-aspartic acid cell attachments sequence. A major role of these proteins is in modulating cell attachment and adhesion and mediating mineralization of the organic matrix.
Gla-containing proteins, represented by osteocalcin, take part in vitamin K–dependent enzymatic reactions. The final grouping of proteins is that of growth factors such as TGF-β, BMPs, insulinlike growth factors, and an ever-growing list of growth factors and their associated binding proteins. These factors, though present in small amounts, are thought to play important roles in bone formation and repair. Specifically, these factors can induce cellular differentiation of mesenchymal cells to osteoblastic lineage. In addition, they have complex interactions locally on the genetic control of repair and development of tissue. In summary, the extracellular matrix of bone is an extremely complex solid-state matrix that interacts with cellular components for the regulation of bone physiology.
Biomechanical Properties
The composite nature of bone affords it unique mechanical properties to support its physiologic role. Bone also has anisotropic properties, meaning different properties along different axes. Measurements of bone strength are subject to various modeling and experimental considerations; however, comparison to steel places it at 10% relative strength. Compression of bone can be achieved because of the structural makeup, which permits force transduction. The result of compressibility is shortening or deformation of the bone; clinically, the response to such compression is resorption, or “creep.” When bone is deformed by elongation, deformation is limited to ∼ 2% before fracturing. The structure of bone, whether compact or cancellous, affects its strength, with cancellous bone having less than 10% the strength of compact bone. Measurements of bone strength have reported cortical bone with a compressive strength of up to 140 MPa and torsional or elastic modulus of 14 GPa. Various pathologic conditions, such as osteoporosis, affect the microstructure of bone and thus the mechanical strength, which in turn affects the potential for fracture.
The craniofacial skeleton has evolved to provide protection to neurosensory structures (brain and eyes) and allow mastication. The structure of the calvarium, midface, and mandible allows for efficient function and protection. The skull and midface perform protective functions; therefore, repairs of these are usually less dependent on mechanical factors during healing.
Force transduction within the mandible occurs at the attachment of the muscles of mastication and at the dental occlusal plane. Model analysis of the mandible reveals that the muscles’ forces are translated maximally at the angle and vertical ramus. The reactive forces generated in the occlusal plane bend the mandible forward, creating zones of tension in the alveolar region. When repairing mandibular disruption, attention to these zones of tension is imperative. Bite forces (up to 700 N) are generated along the occlusal planes. Calculation of the force and force-moment should be considered in the selection of fixation repair with certain minimum load resistance. These considerations are important in the design and placement of fixation devices, particularly in the mandible to maintain active function.
Fractures
Fractures are the result of mechanical overload—that is, failure to resist deformation under loading, resulting in the loss of structural integrity. Detailed analysis of fracture configuration has been done in terms of the force or load, energy release, and specific tissue characteristics. Torque, avulsion, bending, and compression result in specific patterns such as transverse, oblique, impacted, or comminuted. The structural disruption is accompanied by disruption of the osseous blood supply and is dependent on the dynamic nature of the injury disruption of soft tissues and associated neurovascular injuries. The vascular compromise is more significant in the mandible due to the relative thickness of the compact bone component affecting intracortical blood supply. However, the overall abundant blood supply aids in the healing and resistance to infection.
Fracture Healing
Restoration of function and original structure can be understood and evaluated on a biologic and mechanical basis. Most fractures reach mechanical functional healing prior to histologic or biologic restoration of premorbid structure. The basic tenets of wound healing in bone are the requirement for functional cells, adequate nutrition (i.e., vascular supply), and mechanical environment to support the fragment ends in apposition. The initial events result in a process of internal and surface remodeling; increased DNA synthesis and cell proliferation in the subperiosteal region begin almost immediately. Nonperfused bone is replaced by vital bone via remodeling, and proliferating cells secrete osteoid (extracellular matrix), which is then mineralized and forms bone spicules. With progressive healing and osteoclast remodeling, the bone spicules are replaced by mature lamellar bone, and trabecular marrow spaces develop.
Within the extremes of interfragmentary motion, two general patterns of healing are described. This is in fact a continuum; however, it is best to consider the two classically described processes. With limited interfragmentary motion, the conditions for bone formation exist as already described; the contact zones of the fragments are not deformed and permit the osteoclasts in the “cutting cone” to cross the contact zone and connect newly formed osteons, which link the fragments. This is described as primary bone healing or union. Gaps across the contact zone allow for primary lamellar bone formation if immobilization is maintained; larger gaps induce a pattern of woven bone, which is then secondarily remodeled to lamellar bone. Larger but still immobilized gaps reach a critical defect size, which cannot support bone formation conditions and are therefore filled with fibrovascular tissue.
Under conditions of greater interfragmentary motion, the distractive forces in the fracture gap exceed the level of requisite stability necessary for primary healing across the gap. A process or “cascade” of tissue differentiation ensues, with the formation of granulation tissue to connective tissue, fibrocartilage, mineralized cartilage, woven bone, and finally compact bone. This process involving formation of a cartilaginous callus is considered secondary bone healing, or the extraperiosteal reaction to fracture with chondrogenesis and eventual endochondral ossification ( Fig. 60.1 ).

The cellular events are well documented based on experimental studies. Attached muscle and other soft tissues immediately outside of the periosteum show increased activity. Extravasated blood (or hematoma) is noted between the muscle fibers and periosteum. Inflammatory cells are noted in the hematoma (polymorphs and then macrophages). This follows the changes in the adjacent muscle with proliferation of fibroblasts and other cells within the degenerating muscle fibers. The basement membrane of the muscle fibers loses its structural integrity, allowing the fibroblasts to intermix with the hematoma cells and periosteal cells, forming a greater callus mass. The callus mass is then quickly vascularized with formation of small arterioles and venules.
The next stage in the tissue cascade is formation of cartilage within the callus and adjacent to areas of membranous bone formation near the junction between the cortex and subperiosteal bone. The cell proliferation decreases and a basophilic matrix is secreted devoid of blood vessels. The area becomes more avascular and lacunae form around the cells with hypertrophy of nuclei consistent with chondrocytes. Chondrogenesis continues in the same fashion as growth-plate formation during bone formation or maturation. The mature matrix and hypertrophic cells are calcified and invaded by blood vessels from the immediately adjacent membranous bone, then remodeled to woven and ultimately lamellar bone indistinguishable from subperiosteal bone formed via membranous ossification.
Cartilage formation is the primary mechanism of fracture healing in long bones and within the craniofacial region. It is usually seen in the mandible, but is also seen at other sites where conditions do not favor primary bone healing. Clinical conditions, including interfragmentary motion, can affect the local healing response. Diabetes and metabolic insult (chemotherapy), which impair cartilage formation, also play a role. It has been noted that within a given fracture, both types of healing may be noted.
Local regulation of fracture repair has received considerable attention. Growth factors important in local control can be produced by the associated inflammatory cells and found within the bone matrix itself. Osteoblasts, macrophages, or chondrocytes within the callus can also synthesize the factors. Growth factors prevalent in the fracture site are presented in Table 60.1 . What has been initially discerned by various investigations is that TGF-β released from platelets initiates callus formation. Acidic fibroblast growth factor appears to regulate the proliferation of chondrocytes, and as the callus matures, synthesis of TGF-β is increased in the matrix and, along with other factors, induces chondrocyte maturation and initiation of endochondral ossification.
There is significant interplay among these local regulators of bone repair with the BMPs. BMPs are a subdivision of the TGF-β superfamily and play a crucial role in the process of cell growth and differentiation during bone regeneration. There are eight BMPs (2 to 9; BMP1 is a proteinase and is not part of the TGF-β superfamily). When a bone is fractured, pluripotent progenitor cells are activated by the local immune response. The BMPs then bind to membrane receptors on these cells, resulting in activation of the osteocalcin gene, which transcribes a signal protein that eventually leads to differentiation of the cell into an osteoblast. BMP3 (osteogenin) has been localized in perichondrium, cartilage, periosteum, and bone, as well as the membranous bones of the craniofacial skeleton, and has been shown to have the highest bone-inductive activity of all the BMPs. Further elucidation of osseous wound healing will undoubtedly aid in the development of new strategies for fracture repair.
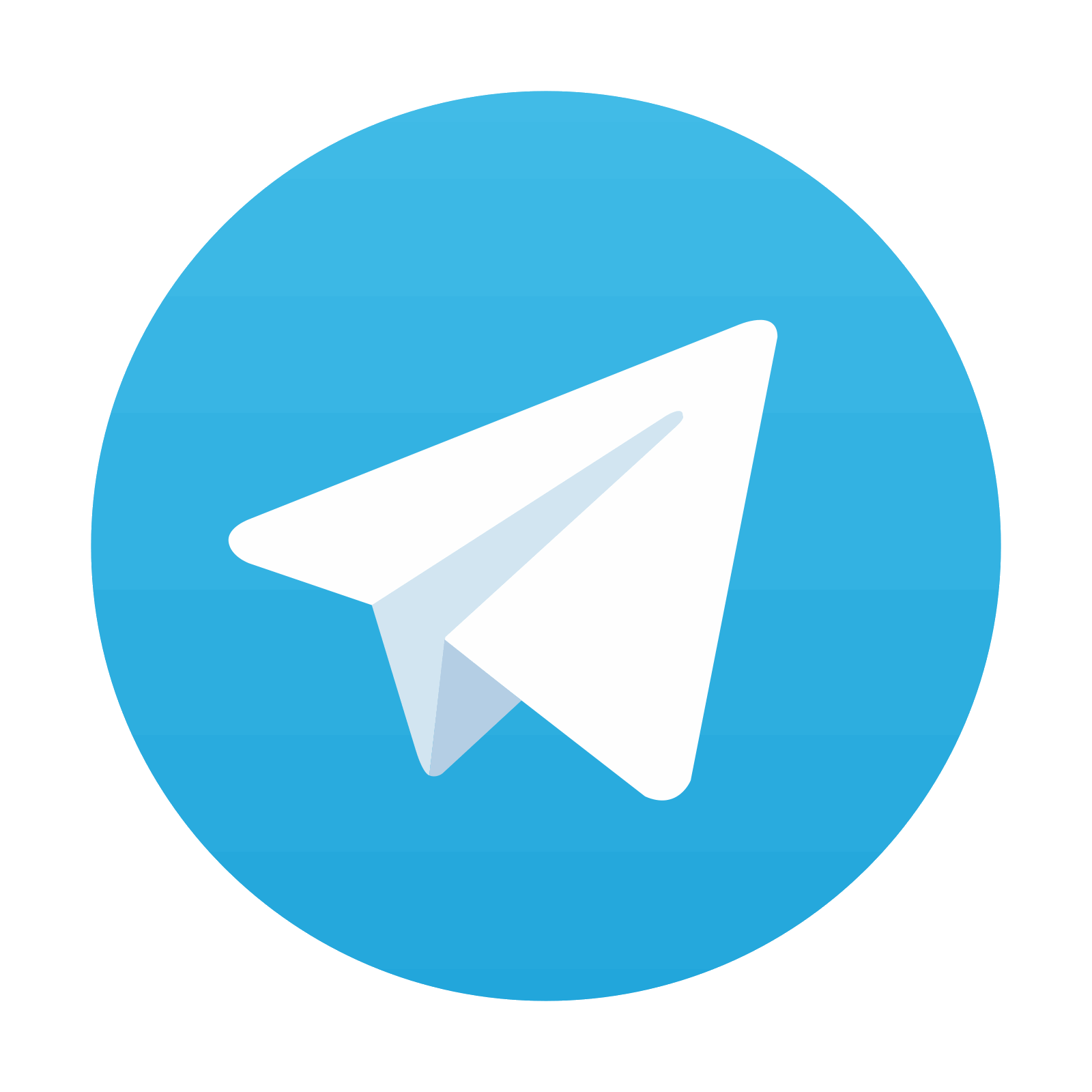
Stay updated, free articles. Join our Telegram channel
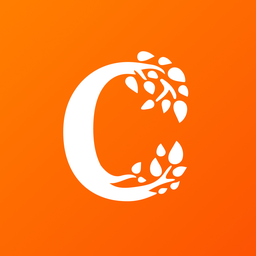
Full access? Get Clinical Tree
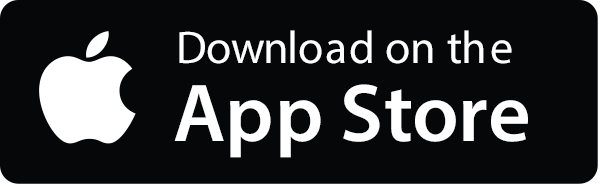
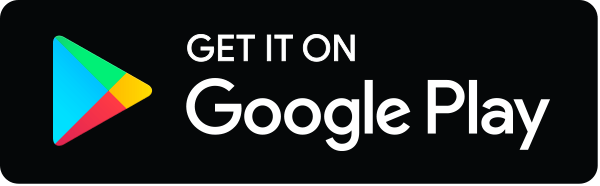
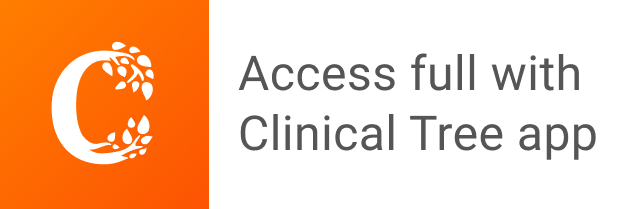