Background
The process of tissue expansion in reconstructive surgery was first reported by Charles Neumann, who described its application for auricular soft tissue reconstruction in 1957. Neumann simply applied concepts found in both normal physiological as well as pathological processes of the human body. Examples of this phenomenon include pregnancy, where the abdomen increases in size to accommodate the enlarging fetus, and rapid neoplastic growth, which may result in soft tissue expansion, deformity, and eventual skin breakdown. African tribes have also adopted tissue expansion as part of their cultural background, enlarging facial structures such as the lip or the ear.
Nearly half a century later, tissue expansion was repopularized by Radovan for use in postmastectomy breast reconstruction, although its applicability soon became evident in other branches of plastic surgery. Tissue expansion enables soft tissue coverage of large defects secondary to burns, trauma, congenital malformations and cancer excision. It involves the insertion of an implant adjacent to a wound or defect that needs to be resurfaced. The implant is then incrementally enlarged by injection of isotonic saline, resulting in stretching of the overlying soft tissue. The expanded skin can then be used to resurface a defect or incorporate permanent prostheses, including those used for postmastectomy breast reconstructions. Tissue expansion exploits the principles of controlled mechanical skin overstretch in order to generate new tissues with ideal compliance and almost perfect skin color, texture match, and sensibility to the resurfaced defect.
However, this useful tool on the reconstructive ladder does not come without its complications. In this chapter, we review the effects of tissue expansion on soft tissues and explore the tenets that govern its safe and effective use in the patient. We also discuss recent developments in tissue expansion such as external volume expansion and needleless expansion techniques with carbon dioxide.
Viscoelastic Properties of Skin
The structural components of skin can be altered by expansion as demonstrated by several biomechanical studies. Its viscoelasticity means that the mechanical response to loading involves both a viscous (time-dependent) and an elastic (time-independent) component. Skin is mainly composed of dermis and epidermis. The predominant proteins in the dermal layer are collagen types I and III, which are produced by fibroblasts. In the relaxed state, their triple helical structure results in a wavy and convoluted alignment. Upon stretching, the collagen fibers straighten and realign parallel to each other. The skin’s elasticity, essential for joint movements, is due mainly to superficial thin bundles of microfibrils associated with progressively larger amounts of amorphous elastin to form elastic fibers. Once strain is released, elastin fibers return collagen to its relaxed coiled posture. If stretched excessively, elastin is prone to fragmentation, resulting in a loss of recoil. Viscoelastic properties change with age and repeated mechanical forces, resulting in skin laxity.
Skin is a dynamic organ and acclimatizes to constant mechanical forces applied to it due to its viscoelastic properties ( Box 6.1 ). On a tissular and cellular level, constant mechanical stress induces changes termed mechanical creep and biological creep . These phenomena interact to restore resting tension of stretched tissues to baseline – stress relaxation ( Fig. 6.1 ). For example, a wound that was under tension intraoperatively but relaxed at outpatient follow-up is an example of stress relaxation.
Mechanical Creep
The elongation of skin under a constant load over time
Collagen fibers stretch out and become parallel
Elastin undergoes microfragmentation
Interstitial fluid is displaced (e.g., during intraoperative tissue expansion and skin suturing under tension)
Biological Creep
The generation of new tissue secondary to a persistent chronic stretching force (e.g., seen during pregnancy)
Stress Relaxation
The tendency for this resistance of skin to a stretching force to decrease when held at a given tension over time

Creep is defined as the stretching of skin under a constant load over time. In order to explain the biomechanics of skin during the process of tissue expansion, a distinction was made between mechanical and biological creep. Mechanical creep describes the clinically observed elongation of skin beyond the original stretch when placed under sudden constant tension and is thought to be attributable to the morphological changes (stretching) of the dermal framework in response to stress. Upon stretching, the formerly convoluted collagen fibers straighten and realign parallel to each other, resulting in the extrusion of fluid from the collagen network and microfragmentation of elastic fibers, thus making the skin more viscous.
Biological creep occurs with the formation of new tissues as a result of a persistent chronic stretching force in an attempt to relieve the stress (stress relaxation). It underlies the mechanism of gap junctional disruptions, which results in cellular proliferation. Several studies have confirmed that the gain in skin surface area after expansion was caused by new skin formation rather than simply stretching the pre-existing skin. Over a period of 6–12 weeks, a multitude of growth factors, cytokines, adhesion molecules, and signal transduction proteins are induced to stimulate epidermal thickening, with concurrent dermal thinning and collagen fibril alignment of the newly expanded tissues ( Figs. 6.2 and 6.3 ). In fact, a study conducted by Vander Kolk et al demonstrated a significant increase in collagen production in expanded skins compared to control skins. Additionally, growth factors, including epidermal growth factor (EGF), transforming growth factor beta (TGF-β), and platelet-derived growth factor (PDGF), are upregulated to stimulate neoangiogenesis of the expanded tissues. Deformational forces acting on the cell membrane and disruption of integrins reduce cell–matrix adhesion and induce conformational changes of membrane proteins. Calcium channels open, leading to signal transduction and cytoskeletal microfilament contraction. The actin cytoskeleton then transmits mechanical forces intracellularly, resulting in increased gene expression and mitosis via interaction with protein kinases, second messengers, and nuclear proteins. Cellular proliferation then results in tissue enlargement.


Enhanced vascularity is among the most noticeable advantages of expanded tissues, exhibiting both higher vessel numbers and larger calibers compared to non-expanded counterparts. Enhanced vascularity is also seen in external volume expansion, , which was initially used by Khouri et al for non-surgical breast augmentation, and using some of the principles of tissue expansion.
Similar to traditional tissue expansion techniques, external volume expansion devices use suction to mechanically stretch the skin. They induce hypoxia, inflammation, and soluble mediators which in turn will result in cell proliferation and angiogenesis. The latter findings have been demonstrated in animal models. The high vessel density given by external expansion techniques has enabled better autologous fat graft take.
Pietramaggiori et al demonstrated that cyclic mechanical forces applied to the skin of living rats stimulated cell proliferation and vascular remodeling. Additionally, a study by Erba et al supported the angiogenic role of vascular endothelial growth factor (VEGF) in cyclic dermal stretching. Stretched skin presented with more densely packed vessels as well as a significantly increased thickening. However, the most important change during tissue expansion is tissue proliferation secondary to stress-induced cellular changes (biological creep). Sheng et al demonstrated that transplantation of angiogenic growth factor-secreting cells into expanding skins of rats resulted in accelerated tissue expansion, enhanced neovascularization of the expanded skin and promoted cell proliferation. Expression of EGF, VEGF, and β-FGF were significantly enhanced in treated animals compared to control animals. Growth factor supplementation may well play an important role in the future of tissue expansion, both in reducing hospitalization times and flap retraction after expansion.
Tissue Changes during Expansion Techniques
In order to fully understand the mechanics involved in tissue expansion, we will first familiarize ourselves with the structural components of skin. Skin is a multilayered structure and has three well-defined anatomical regions. The outermost layer, the epidermis, forms a thin, uninterrupted and protective layer across most of the body’s surface but contributes little to the skin’s mechanical properties. The bottom layer, the hypodermis, is mainly made of fatty tissues and serves as an insulating layer. The middle dermal layer holds most of the skin’s adnexae, including nerve endings, sweat, and oil glands and consists of three main structural components that underlie the skin’s strength and elasticity: collagen, elastic fibers, and extrafibrillar matrix. The dermis can be further divided into papillary and reticular layers. The papillary dermis contains thin collagen fibrils which are packed together into thicker collagen fibers ( Fig. 6.4 ). Only about 15% of the dermis is type III collagen, most of which is found in the papillary layer. Collagen type I, the most abundant building block in vertebrates, is the principal protein providing tensile strength to the skin.

Johnson et al assessed the effects of tissue expansion on the mitotic activity in the epidermis in order to address the question whether there is a net gain in epidermal tissue. Their studies confirmed that tissue expansion caused a marked increase in epidermal mitotic activity in a guinea-pig model. Studies on human volunteers supported this finding and demonstrated a significantly thickened epidermis of expanded human skin compared to non-expanded skin. Although expanded tissues maintain their phenotypic characteristics, mechanical strain induces an increase in DNA synthesis and therefore cellular proliferation. Biochemical pathways involved in this process include cytoskeletal, extracellular enzyme, membrane and cytosolic components that converge together to convert the pathway of mechanotransduction seen in tissue expansion into a cellular response, ultimately leading to cellular proliferation. The dermis, in contrast, becomes thinner upon stretching, with hair follicles moving further apart. It may take up to 2 years for the thickness of this layer to return to normal.
Subcutaneous fat tissues were also found to thin after expansion. Adipocytes were flattened, surrounded by fibrosis, or disappeared altogether during the expansion process. Similarly, skeletal muscles are known to undergo atrophy after expansion resulting in the so-called “bath-tub depression.” Histologically, the atrophic muscle cells are surrounded by fibrous tissue and demonstrate abnormally arranged sarcomeres, large amounts of sarcoplasm, and also numerous large mitochondria. As opposed to the changes in muscle tissues, the loss of subcutaneous fat is permanent.
Enhanced angiogenesis in expanded tissues versus non-expanded counterparts is of clinical significance and presents an advantage in flaps raised on such skin. Improved survival of flaps raised on expanded skin shows properties similar to a delay phenomenon. This was confirmed by Cherry et al, who, using a pig model, elevated random pattern flaps in expanded tissues or non-expanded tissue to determine whether these flaps survived for the same time intervals. Flaps raised on expanded tissue had the greatest increase in surviving length, averaging 117% over control flaps, which was in large part due to an increased vascularity as well as vasodilatation in the angiograms of skins expanded over a period of 5 weeks. The pressure and mechanical stresses applied to expanding tissues resulted in tissue hypoxia, which induced the formation of an increased microcirculation in expanded tissues. Increased vascularity is thought to result from increased gene expression and significantly higher levels of angiogenic factors such as VEGF in expanded tissues compared to non-expanded controls.
Rapid rates of expansion have further been demonstrated to increase flap microvascularity compared to slowly expanded tissues. However, it should be noted that rapidly expanded tissues may become profoundly hypoxic up to 48 hours, which may compromise flap survival. In addition, significant patient discomfort accompanying rapid tissue expansion may limit the rate of expansion.
External volume expansion has broadly similar effects on tissues compared to conventional tissue expansion. Lancerotto and colleagues, in a murine model, demonstrated that the application of controlled non-invasive suction results in cell strain which in turn gives way to cell proliferation. Based on their results, after 48 hours of external volume expansion four factors, which include mechanical stimulation of cell, edema, and hypoxia, all contribute to cell proliferation in the epidermis and dermis as well as angiogenesis. Chin and colleagues have also demonstrated in a mouse model that external volume expansion provides ischemic preconditioning of tissues via hypoxia/ischemia of tissues.
Evolution of Tissue Expansion Devices
Each tissue expander needs to be carefully selected for its desired purpose, making tissue expansion a highly individualized procedure. Depending on the size and shape of the defect to be covered, different expander types may be selected ( Fig. 6.5 ). The basic design is an inflatable silicone elastomer reservoir. Isotonic saline is delivered in a controlled fashion via a valve port system which is either integrated into the expander or connected to the prosthesis via silicone tubing ( Fig. 6.6 ). An integrated system offers the advantage of undermining a single pocket for expander placement, but also places the implant at risk of perforation by a misplaced needle. Internally placed remote ports remove the danger of expander perforation, but introduce potential complications, including kinking of the port, tube obstruction, and migration. To avoid these complications, the undermining for the port pocket is minimal, the port is placed over firm, supporting tissue and, if need be, the port position can be fixed with sutures. Externally placed portals have proved particularly useful in the pediatric population as they may alleviate pain and anxiety associated with frequent injections and the safety of such ports has been demonstrated repeatedly.

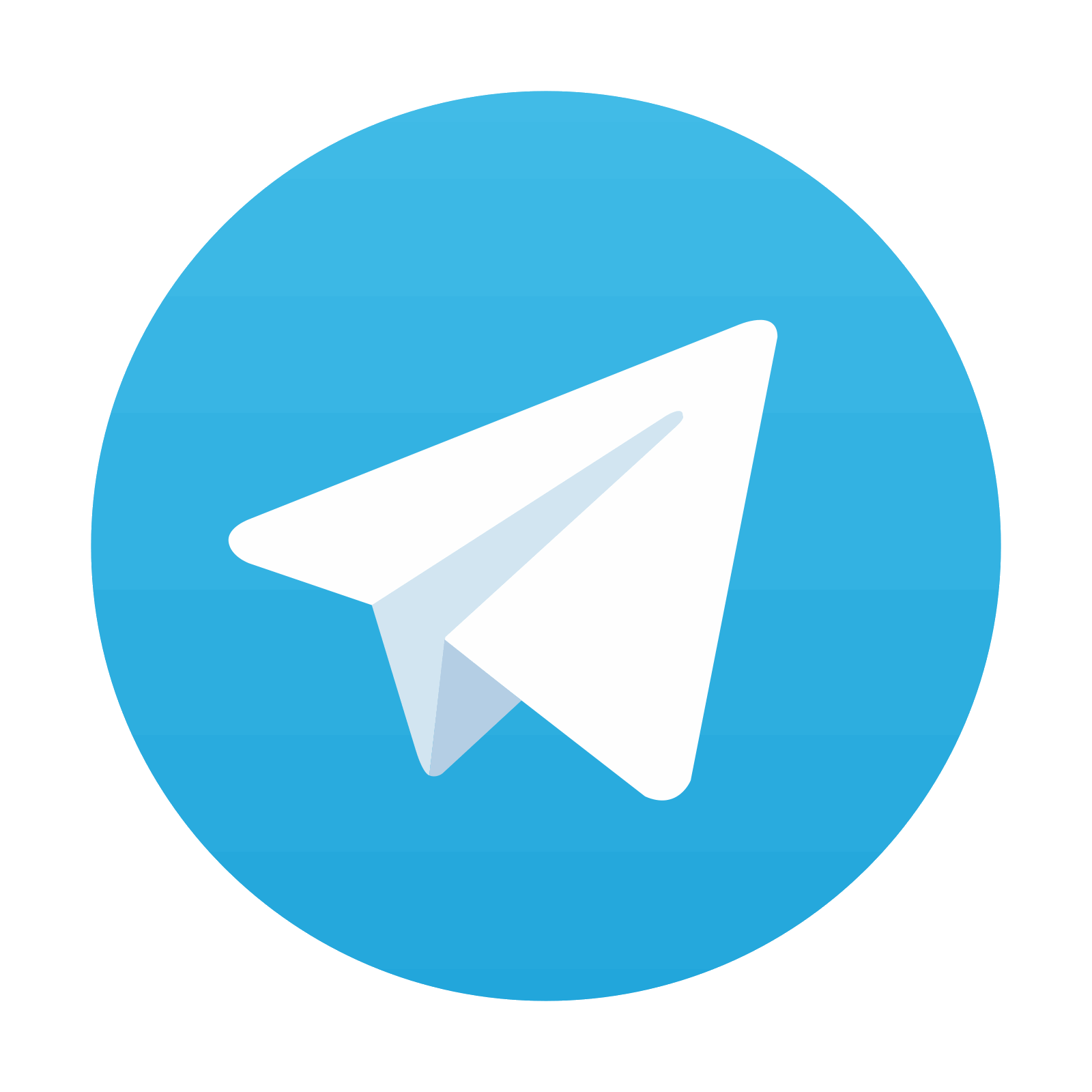
Stay updated, free articles. Join our Telegram channel
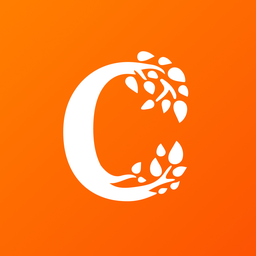
Full access? Get Clinical Tree
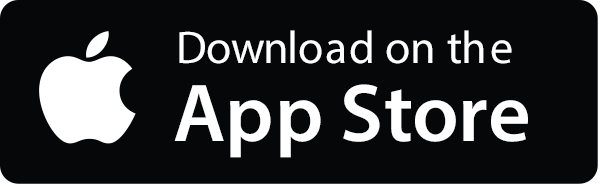
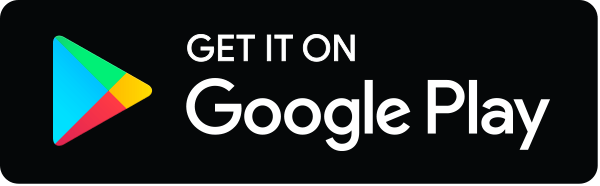
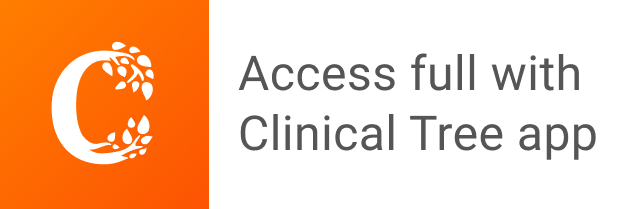