Fig. 3.1
Wet technique liposuction in the subcutaneous abdominal adipose tissue of a patient. (a) Surgeon harvesting fat with the liposuction cannula. (b) Stromal vascular fraction containing ASCs is selected from the lipoaspirate. (c) Total volume of stromal vascular fraction after liposuction. (d) Commercially available water-assisted liposuction device for ASCs isolation
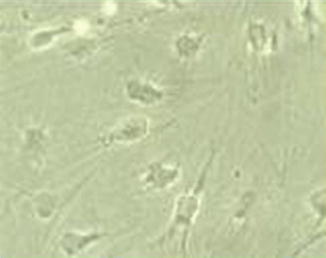
Fig. 3.2
Tissue culturing of ASCs. ASCs under 40-fold microscope magnification (unstained)
3.6 Supportive Matrix Material
There is increasing evidence that the differentiation of stem cells is highly dependent on the extracellular microenvironment including various matrix proteins and growth factors that facilitate proliferation and consecutive cell differentiation [33–35]. Accordingly, numerous strategies have been employed ranging from biologically derived scaffolds such as fragmented omentum, MatrigelTM, collagen, fibrin, polycaprolactone, gelatin, and hyaluronic acid to various synthetic scaffold materials, including poly(lactic-co-glycolic) acid (PLGA) and PLGA-polyethylene (PLGA-PEG) scaffolds, some of them designed as injectable biodegradable gels or beads for molecular delivery [34–51] (Tables 3.1 and 3.2) (Fig. 3.3). There is frequent reporting on the adipogenic properties of Matrigel, a protein mixture secreted by mouse sarcoma cells. Distributed as commercially available base membrane, this substrate has shown to be advantageous for the differentiation of adipose precursor cells when injected subcutaneously into nude mice [34, 52]. However, the main effect of Matrigel seems to be the induction of angiogenesis. Further, Matrigel has been tested for its properties for adipose tissue engineering in combination with various growth factors and membrane proteins [53–56]. Although Matrigel has proven utility compared to other scaffold materials of natural or synthetic origin, its broad application in clinical practice is problematic because of its tumor-derived xenogeneic origin [49].
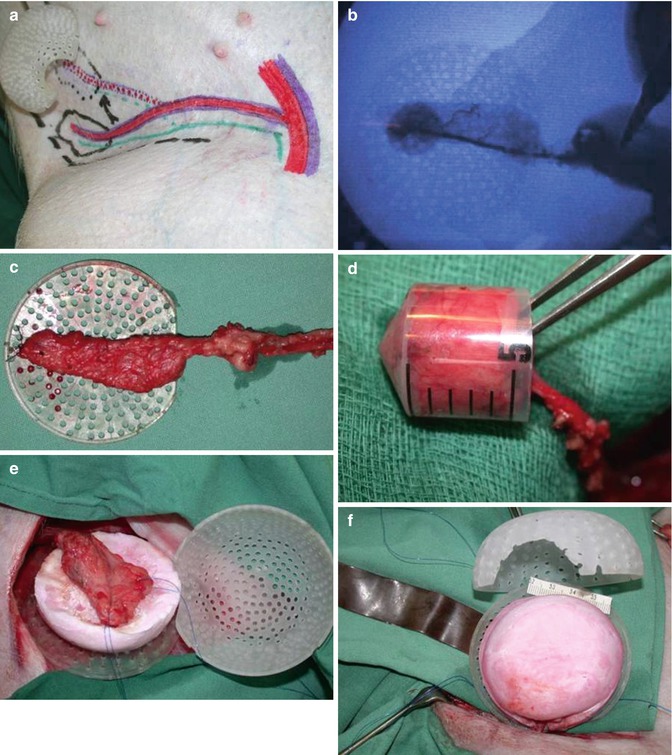
Table 3.1
Biomaterials for vascularized adipose tissue engineering
Scaffold/matrix material | Hypothesis | Method | Model type | Results/conclusion | Authors |
---|---|---|---|---|---|
MatrigelTM | Proliferation and maturation of adipocyte precursor cells depends on microenvironment | Matrigel supplemented with bFGF | S.c. injection into 6-week-old mice | Adipose tissue growth until 3 weeks after the injection with a persistence of at least 10 weeks | Kawaguchi et al. [34] |
Collagen | Maturation and vascularization of preadipocytes is enhanced when seeded onto collagen sponges with uniform pore size and regular lamellar structure | Preadipocytes seeded onto collagen sponges | S.c. implantation into immunodeficient mice | Adipose tissue and a rich vascularization adherent to the scaffolds under a capsule | von Heimburg et al. [36] |
Collagen/gelatin | Controlled release of basic fibroblast growth factor (bFGF) of gelatin microspheres enhances adipose tissue formation | Gelatin microspheres containing (bFGF) | S.c. injection into nude mice | Gelatin microspheres enabled preadipocytes to induce adipose tissue formation | Kimura et al. [37] |
Extent of adipose tissue formation was dependent on the number of preadipocytes and the bFGF dose | |||||
Fragmented omentum | Omentum produces high level of vascular endothelial growth factor (VEGF) | Utility with or without cotransplantation with preadipocytes | S.c. injection into Wistar rats | VEGF production were observed in transplanted tissues 12 weeks postoperation | Masuda et al. [38] |
Cotransplantation with preadipocytes enhanced adipose tissue formation significantly | |||||
Hyaluronan benzyl ester (HYAFF®)/hyaluronic acid (HA) | Larger pore structure (400 μm vs. 40 μm) and coating of HYAFF® sponges with HA are beneficial for distribution, proliferation, vascularization, and adipose tissue formation | HYAFF® seeded with hASC plain or coated with the HA | S.c. implantation into athymic nude mice | Penetration of cells into both types of scaffolds, with more extensive formation of new vessels throughout the construct but with only minor adipose tissue | Hemmrich et al. [39] |
Vessel formation was not influenced by HA coating | |||||
Sufficient differentiation of hASC was observed neither in coated nor in uncoated sponges | |||||
Fibrin | Injection of hASCs in fibrin glue as a transport vehicle human adipose tissue engineering | Different concentrations of undifferentiated hASCs were suspended in fibrin or fibrin glue injected alone | S.c. injection into athymic nude mice | Volume and shape of implants with hASCs remained stable. Implants without cells were completely resorbed within 3 weeks | Torio-Padron et al. [40] |
Alginate | Predifferentiated ASCs mixed with alginate systems form ectopic adipose tissue in vivo | After 10 days induction in vitro, predifferentiated ASCs were seeded in an alginate gel system and implanted subcutaneously | S.c. implantation into nude mice | Adipose tissue constructs maintained two-thirds of their original size after 8 weeks of implantation | Jing et al. [41] |
Gel consisting of basement membrane proteins and growth factors vs. Matrigel™ | Gels consisting of isolated proteins from a natural subcutaneous adipose tissue induce differentiation of preadipocytes in vitro and vascularized adipose formation in vivo | Gels or Matrigel™ in silicone chambers surrounding the epigastric pedicle | Epigastric implantation into rats | Gels caused preadipocyte aggregation and differentiation in vitro and vascularized adipose formation in vivo. Adipose tissue formation was significantly greater compared to Matrigel™ | Uriel et al. [49] |
Placental decellular matrix (PDM)/cross-linked HA (XLHA) | Assessment of PDM-based biomaterials on hASC adipose tissue formation | PDM and PDM combined with XLHA seeded with hASC | S.c. implantation into nude athymic mice | Both scaffolds maintained three-dimensional volume and supported mature adipocyte population | Flynn et al. [35] |
XLHA positively affected angiogenesis and adipogenesis | |||||
No positive effect of predifferentiation in vivo | |||||
Effects of predifferentiation of hASC prior to implantation | |||||
Decellularized extracellular matrix (DECM) | DECM isolated from porcine adipose tissue as a xenogeneic biomaterial for tissue engineering | A decellularization was used for extracting an intact ECM while effectively eliminating xenogeneic epitopes and minimally disrupting the ECM composition | S.c. injection of ECM into mice | DECM exhibited biocompatibility, long-term stability, and bioinductivity in the analysis if histological sections and adipogenic-specific genes | Choi et al. [42] |
Table 3.2
Synthetic scaffold materials for vascularized adipose tissue engineering
Scaffold/matrix material | Hypothesis | Method | Model type | Results/conclusion | Authors |
---|---|---|---|---|---|
Poly (lactic-co-glycolic) acid (PLGA) | Long-term maintenance of adipose formation within a model polymer-cell system | PLGA seeded with rat ASC | S.c. implantation into Lewis rats | Maximum adipose formation at 2 months, followed by a decrease with complete absence of adipose tissue and PLGA at 5–12 months | Patrick et al. [43] |
The long-term maintenance of adipose tissue | |||||
PLGA-polyethylene (PLGA-PEG) | (PLGA-PEG) microspheres as drug delivery vehicles for bFGF, insulin, or insulin-like growth factor-1 (IGF-1) improve free fat graft growth | (PLGA-PEG) microspheres together with autologous free fat graft | S.c. implantation into Lewis rats | Fat graft volume was most enhanced by insulin or solely IGF-1 or the combination of both | Shenaq and Yuksel [44] |
PLGA/fibrin | Mechanical support structures of poly(glycolic acid) fiber-based matrices with poly(L-lactic acid) enhance volume stability of engineered adipose tissues | hASC suspended in fibrin matrix | S.c. injection into pockets of athymic mice | Volume-stable adipose tissues can be engineered in vivo using mechanical support structures | Cho et al. [45] |
PLGA | PLGA scaffolds produced by two different technologies—thermally induced phase separation (TIPS), solvent casting and particulate leaching (SCPL) were compared | In vivo results were based on a microsurgically created arteriovenous (AV) loop sandwiched between two TIPS scaffolds placed in polycarbonate chamber | S.c. implantation into groins of rats | Foreign body response and reduced vascularity was evident histologically between 2 and 8 weeks in TIPS scaffolds | Cao et al. [46] |
Tissue death occurred at 8 weeks in the smallest pores. Morphometric comparison of TIPS and SCPL scaffolds indicated slightly better tissue ingrowth but greater loss of scaffold structure in SCPL scaffolds | |||||
Polycarbonate/PLGA | Tissue growth of pedicled flaps within chamber spaces is spontaneous without the enhancement of extracellular matrix | Pedicled flaps in either perforated or non-perforated polycarbonate chamber spaces with or without PLGA scaffolds within the chamber | Sprague–Dawley rats | Adipocyte volume was maximum in chambers without PLGA | Dolderer et al. [47] |
Volume and vascularization were improved in perforated chambers with or without PLGA | |||||
PLGA had no influence on vascularization | |||||
Poly ethylene glycol (PEG) | Biophysical and bioactive approaches to induce vascularization in adipose tissue grafts | (PEG) in four configurations: PEG alone, PEG with basic fibroblast growth factor (bFGF), microchanneled PEG, or both bFGF-adsorbed and microchanneled PEG | S.c. into immunodeficient mice | No neovascularization occurred in PEG alone, but substantial angiogenesis in bFGF-adsorbed and/or microchanneled PEG | Stosich et al. [48] |
Bioactive cues and/or microchannels promote the genesis of vascularized adipose tissue phenotype | |||||
Polycaprolactone (PCL) | PCL maintains the volume of regenerated adipose tissues | Human adipose-derived stromal cells (hASC) seeded on PCL compared to fibrin | S.c. implantation into athymic mice | Volumes/adipose tissue areas of hADSC-PCL scaffold implants were significantly larger than those of hADSC-fibrin implants | Lee et al. [50] |
Adipogenic gene expression increased in hADSC-PCL scaffold implants | |||||
PCL/polyurethane | PCL and polyurethane for their potential use in adipose tissue regeneration | Both scaffold types were seeded with hASCs | Nude mice using a femoral arteriovenous flow-through vessel loop | In vivo angiogenesis and adipose tissue formation were observed throughout both constructs after 2 and 4 weeks, with angiogenesis being comparable in seeded and unseeded constructs | Wiggenhauser et al. [51] |
Fibrous tissue formation and adipogenesis were more pronounced on polyurethane foam scaffolds than on polycaprolactone prototyped scaffolds | |||||
In conclusion, both scaffold designs can be effectively used for adipose tissue engineering |
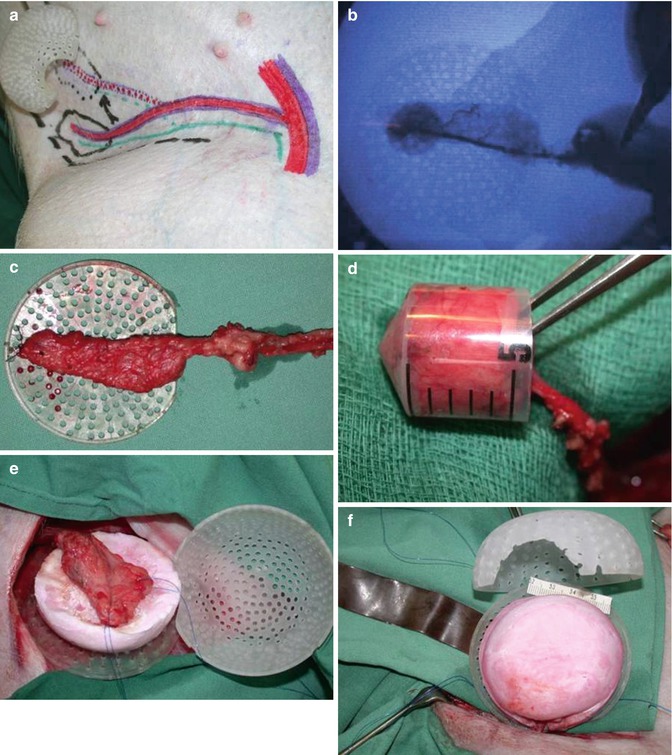
Fig. 3.3
Insertion of adipose tissue with vascular pedicle into chamber space together with PLGA scaffold. (a) Adipose tissue flap with vascular pedicle from the superficial iliac circumflex artery. (b) Angiography of pedicle perfusion. (c) Vascularized adipose tissue with vascular pedicle. (d) Volume assessment (5 mL). (e, f) Insertion of adipose tissue flap in between PLGA scaffold surfaces
The use of naturally derived scaffold materials such as placental decellular matrix and cross-linked hyaluron seeded with ASCs has shown promising effects on adipose tissue formation. Nevertheless, some investigators have noted a fibrous capsule surrounding these constructs in vivo, suggesting some degree of immunoreaction toward the scaffold material [35].
Other approaches have been based on the specific release of growth factors into the repair region. In particular, fibroblast growth factor (FGF) demonstrated a capacity to induce adipogenesis. Studies that investigated the effects of FGF-releasing microspheres or alginate microbeads reported an increase in vascular density, but varying results in the extent of adipose tissue formation [57, 58]. Recently, Uriel et al. attempted to avoid foreign body reactions in the context of wound repair by using a mixture of isolated proteins comprising mainly base membrane components such as laminin, collagen IV, nidogen, and fibronectin derived from subcutaneous adipose tissue. The application of this protein cocktail revealed adipose tissue formation that was enhanced compared to that obtained using Matrigel [49]. Although mostly minor volumes are achieved by the implementation of growth factors and matrix materials, some of these might still have potential clinical utility in scenarios requiring filler materials for low-grade tissue defect sites. On the other hand, the in vivo use of growth factors and potential foreign body materials will indeed be critically scrutinized before eventual translation into widespread clinical practice.
3.7 Vascular Supply
Mature adipocytes are characterized by their essential dependence on a sufficient microvascular system for adequate nutrient supply [59]. The low tolerance toward ischemia is evidenced by the fact that in native adipose tissue, each individual adipocyte is in contact with at least one capillary [33]. Nevertheless, to date no clinical tissue engineering concept provides the possibility for extrinsic vessel anastomosis. Prolonged tissue construct survival in animal models has been achieved by inserting extrinsic vessels into engineered adipose tissue [60, 61]. Although this approach showed promising results and emphasized the close relation between adipose tissue growth/survival and vascular supply, this procedure has not yet been introduced into clinical practice.
Mian et al. were among the first to demonstrate that the presence of a simple vascular system is essential for de novo tissue formation [62]. Furthermore, this group investigated the relation between the type of vascular supply and potential tissue growth for the purpose of skin engineering in vivo. The findings revealed that a ligated arteriovenous (AV) bundle construct is superior to an AV shunt or a flow-through-type vessel formation, with respect to tissue growth and neovascularization [63]. Secondary studies confirmed these observations for adipose tissue [64]. In contrast, larger volumes of novel tissue were reported in groups with an AV shunt-type formation [63] (Fig. 3.4). A higher rate of fibrin extravasation and/or simply vasodilatation due to a comparably increased blood flow within the shunt system has been proposed as contributing to this observed relative increase in tissue volume [63, 64].
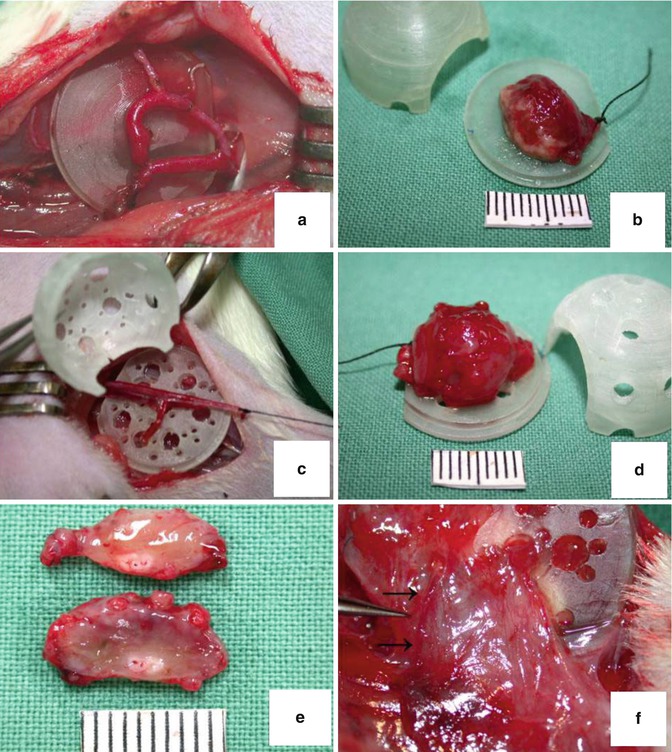
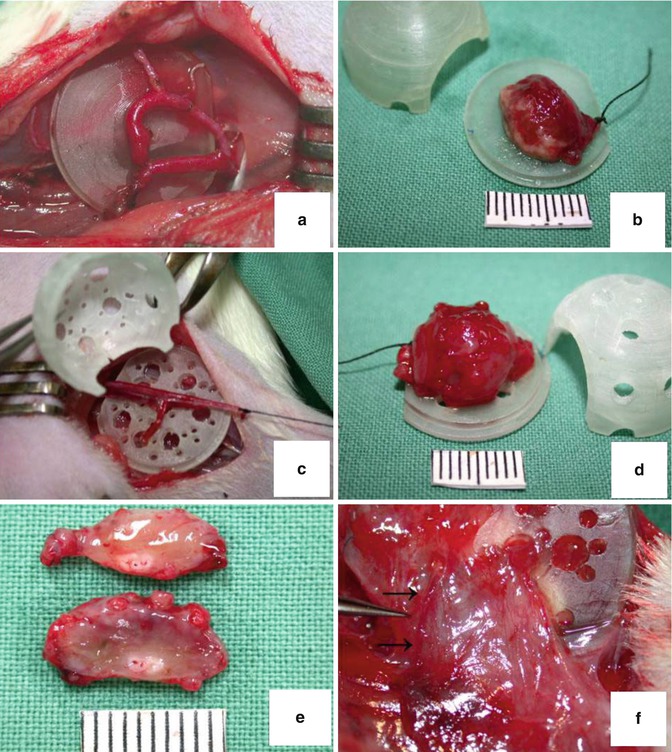
Fig. 3.4
Different types of vascular supply for chamber space tissue engineering. (a) AV shunt type with non-perforated wall chamber at day 0. (b) Engineered tissue constructs at 4 weeks postimplantation. (c) Ligated AV bundle construct in perforated chamber type day 0. (d, e) Engineered and vascularized tissue with removed chamber shell and sectioned without chamber space. (f) Arrows indicate ingrowth of extrinsic vessels
3.8 Chamber Space
Chamber systems consist of an extracellular rigid polycarbonate or silicone scaffold for in vivo tissue growth. The chamber construct is implanted together with an adipose tissue flap, which is inserted into the dome-shaped chamber lumen. The chamber environment not only provides space for novel tissue formation but is also thought to create a hypoxic state and further a protective barrier for fibrin exudate. Both fibrin and hypoxic conditions are reported to be favorable in the case of neoangiogenesis [65, 66
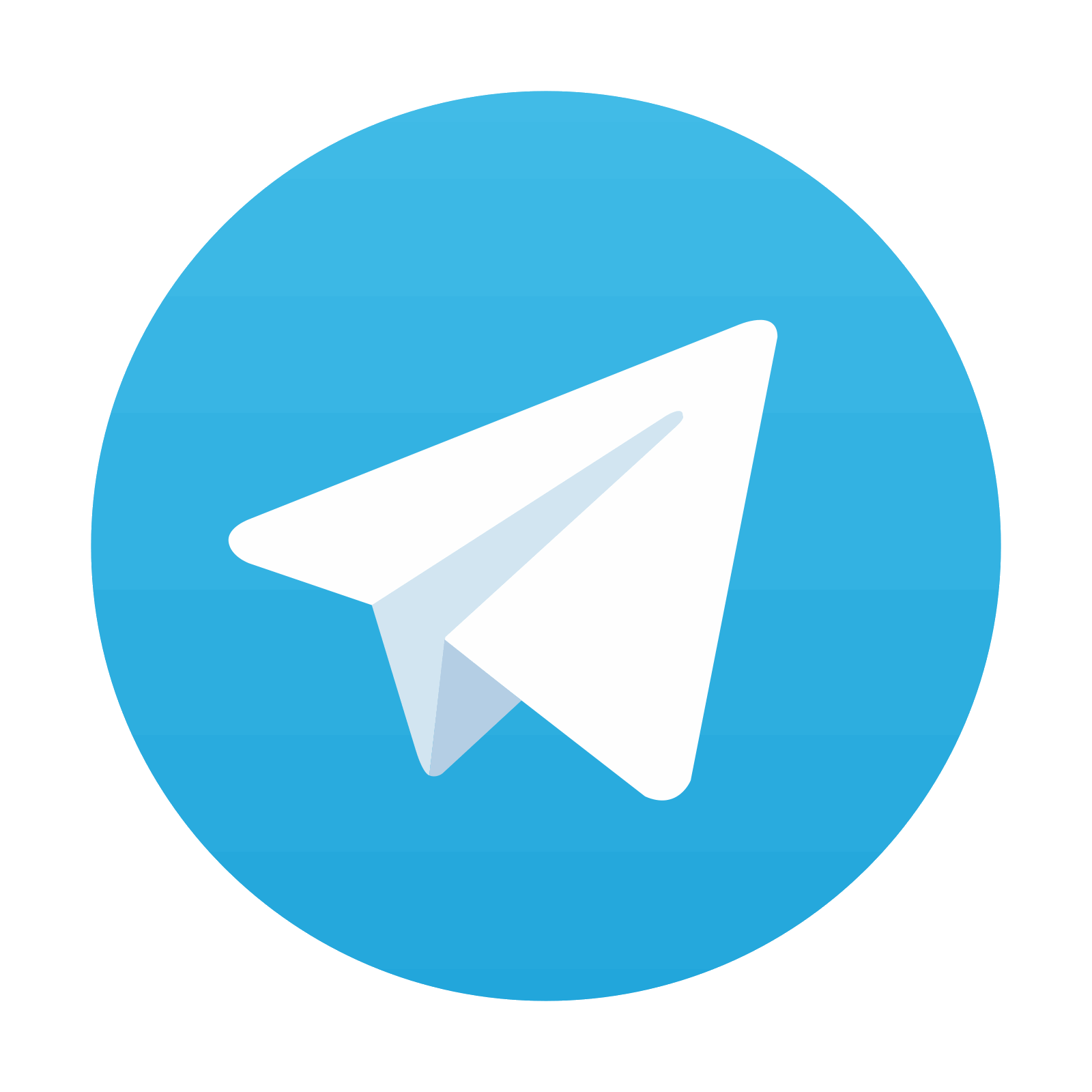
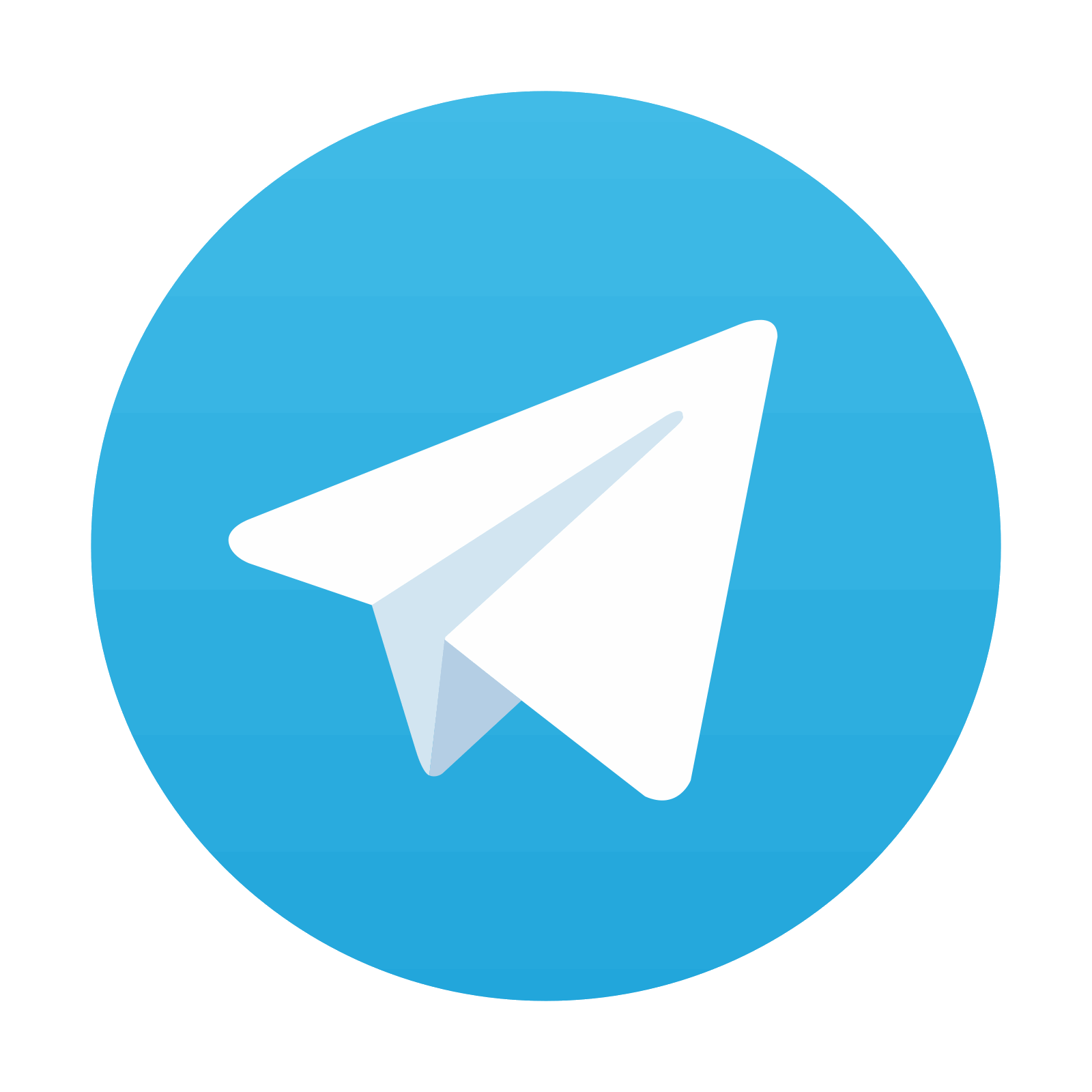
Stay updated, free articles. Join our Telegram channel
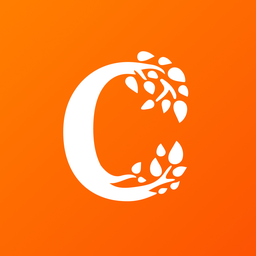
Full access? Get Clinical Tree
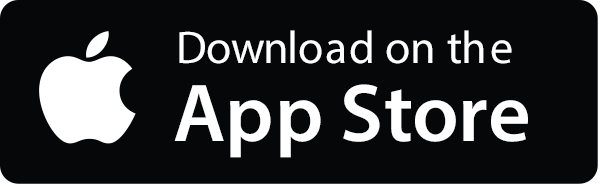
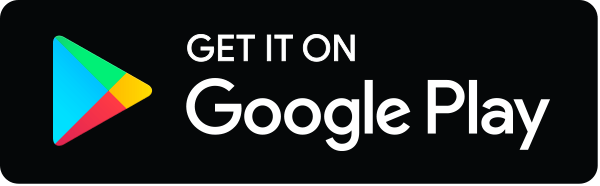