19 Tissue engineering
Synopsis
Tissue engineering, i.e., a growth of three-dimensional (3D) tissues using cells, scaffolds, and blood supply, calls on the skills and expertise of cell biologists, engineers, and clinical surgeons.
It aims to replicate the embryological process of tissue regeneration rather than adult healing and, given the complexity of the process, much of our efforts to date may seem simplistic.
Nevertheless, much progress has been made across the full spectrum of the research field. This chapter outlines the historical beginnings of tissue engineering and discusses the current status, addressing the three structural components of tissue: cells, matrix, and vascular supply.
Biomaterials science and applications are discussed under matrix.
Much of the work in tissue engineering has been performed ex vivo and unfortunately many of these experiments do not predict the fate of cells and matrices in vivo.
Models of in vivo tissue engineering are discussed along with the progress in specific fields.
Very few clinical trials have been undertaken, controls or placebo groups are difficult to incorporate in trial design, and tracking the fate of implanted cells is difficult in humans.
All this needs to be viewed with the perspective that, to date, very few truly tissue-engineered products have come to market despite huge expectations and investments.
Introduction
Definition of tissue engineering
The definition of tissue engineering quoted by most authors is that it is “an interdisciplinary field that applies the principles of engineering and the life sciences toward the development of biological substitutes that restore, maintain, or improve tissue function.”1 It stressed the key roles of engineering techniques, material science, biochemical expertise, and cell biology. What it did not stress was the role that blood supply would play if and when such engineered tissues were to be transplanted into the body. It is to this piece of the tissue-engineering puzzle that plastic surgeons may hold the key and why the clinical application of tissue engineering will become the province of the plastic surgeon.
Regenerative medicine
The classical paradigm of tissue engineering followed Jack Burke’s artificial dermis skin model of seeding cells on to a biodegradable matrix scaffold and implanting it into a vascularized bed.2 This would rapidly nourish the graft and, by a combination of survival of implanted cells and substitution, the “new skin” would become functional. Clinical needs for bone and cartilage determined early research directions and this gave pre-eminence to the engineering materials side of the tissue-engineering pendulum as structural load, stability and ideally biodegradability were clearly essential. The spectacular image of the “human ear” in the mouse (see Fig. 20.22) galvanized scientists and the public alike as to the potential offered by tissue engineering and reinforced the dogma of expanding cells in culture, seeding them ex vivo into scaffold matrix and implanting the composite into the body.3 The journal Tissue Engineering was established in 1996 and many publications focused on novel synthetic biomaterials attempting to replicate pore size and connectivity of nature’s extracellular matrix (ECM) using sophisticated machinery and mathematical modeling. Toxicity, structural integrity, biodegradability, surface modifications, cell survival, adherence, migration, and proliferation could all be tested in ex vivo models. Bioreactors could greatly expand cell numbers and mimic in vivo physical environments to allow more sophisticated ex vivo experimentation.
The ex vivo paradigm stumbled when attempts were made to translate advances into the living animal. Biomaterials proved toxic to cells in some cases and acted as foreign bodies.4 Their dynamic structural behavior was very different to ex vivo testing and cells found life hostile in the new world. Despite the obvious need for multidisciplinary cooperation, most early research was directed in isolation and this has led to delayed progress in tissue engineering. The engineer’s mind set is focused on scaffold structure, tensile strength, biocompatibility, and surface modification while the cell biologist’s is on cell identification, expansion, and differentiation. Scaffold modeling was based on sophisticated replication of human ECMs with respect to pore size, but evaluation was largely done in vitro. Both teams are laboratory-based, observing cell responses outside the living animal, and had limited access to a practicing clinician’s observations, especially plastic surgeons, with respect to the behavior of foreign bodies in vivo. Furthermore, surgeons know from bitter experience with skin grafting that cells seeded on to an implanted scaffold ex vivo and then implanted are unlikely to connect to a blood supply in sufficient time to avoid ischemic death. Vascularization is vital to cell survival and is a key to any successful multidisciplinary partnership towards clinical translation of tissue engineering. Unfortunately, early tissue engineers were beguiled by selecting tissues that were very thin, such as skin, which could rapidly connect to a blood supply or tissue that did not need a blood supply, namely cartilage. Once 3D soft tissues were attempted such as adipose, muscle, or organs, it became evident that cell survival was poor and that blood supply was paramount.5–7
Embryology
Tissue engineering at its core is an attempt to replicate embryology or fetal healing. Here, new tissues form in response to certain signals, both biochemical and biomechanical.8 The tissues are determined or patterned according to a genetically guided time sequence where growth factors and their chemical gradients as well as changing physical forces in the matrix attract, guide, and differentiate primitive cells into functional clusters. In this process, cells will link with a capillary bed, lay down their own matrix, and create an environment appropriate to their expansion and development. This relatively new concept of “self-assembly” is important to appreciate when translating embryogenesis into a model for tissue engineering. As cells change their phenotype, for example, from precursor to differentiated or from motile and proliferative to resting and clustered, their ECM requirements alter accordingly. Many cells secrete their own matrix suitable to their needs. This constant change cannot easily be replicated in the laboratory and it is simplistic to believe we can seed cells on to one specific matrix and assume this will be appropriate for the cells’ needs throughout their development in vivo, nor is it easily conceivable that “smart surfaces” can be imprinted on synthetic matrices in layers programmed to be released according to the changing cells’ needs when at this point we do not know what these needs are.
Examples from nature
A salamander regrows its legs, the fetus repairs wounds with minimal scarring, and humans have the capacity to gain and lose adiposity rapidly. Ruptured tendons can regenerate across gaps when their ends are retained within their synovial sheath where their matrix and cellular environment are maintained and axial mechanical force signals are transduced into biochemical stimulation (Fig. 19.1). Muscles also will regenerate across gaps in the same way.
Historical perspective
The term “tissue engineering” was coined at a meeting sponsored by the National Science Foundation in 1987. Implied in the definition of tissue engineering is the use of living cells, biomolecules, and/or biomaterials.9 One of the earliest human applications of the concepts of tissue engineering is in oral surgery with what it is termed “guided bone regeneration” (GBR) or guided tissue regeneration (GTR).10 This surgical procedure utilizes barrier membranes to direct growth of bone and soft tissue and is predominantly applied in the oral cavity to support new bone growth on alveolar ridges to allow stable insertion of implants.11 The membrane which is inserted under the mucoperiosteum creates a dedicated space that collects the raw ingredients of osteogenesis – periosteal and bone-derived osteoblasts, vascular cells, tissue fluid, and matrix secretions appropriate for the biochemical signaling of bone formation. The rigid surface of a membrane induces biomechanical signals to the cells to proliferate and migrate along its surface. Platelet-enriched plasma is sometimes added to enhance tissue growth by augmenting the growth factor profile.12
Similar phenomena are observed with “distraction osteogenesis,” where long bones are osteotomized within their periosteal sleeve and slowly distracted (Fig. 19.2). The expanding space spontaneously fills with new bone. Just as in GBR, the environment within the newly created space is exclusively conducive to bone formation. Both GBR and distraction osteogenesis are examples of what would now be called endogenous or intrinsic tissue engineering, where the body grows its own tissue in response to local signals of trauma, ischemia, inflammation, and mechanical forces which promote vascularization followed by specialized tissue regeneration.
Cancellous bone grafting, first described by the New Zealand plastic surgeon Rainsford Mowlem,13 is an even earlier example of a tissue-engineering concept, in this case using the extrinsic principle where a scaffold loaded with cells is implanted into a new site. Most of the cells probably die but the bone matrix survives and acts as a scaffold with the appropriate signals to attract new blood vessel and bone-forming cells into its interstices and replace it by “creeping substitution.” The bone remodels according to the biomechanical forces of its new environment, as articulated by Wolf’s law. Bone regeneration has also been encouraged by the implantation of synthetic porous scaffold materials such as Proplast and more recently MEDPOR, usually primed with autologous blood to attract ingrowth of endogenous tissue.
Components of tissue engineering
Cells
Cell sources
Cells used for tissue engineering may be autologous, heterologous, or xenogenic and each type may be mature differentiated or in precursor stem cell form. Embryonic stem (ES) cells are totipotent, infinitely proliferative, and can be differentiated into all tissue types but they are unstable and form teratomas. Adult stem cells are multipotent and limited in their proliferation capacity and differentiation. Originally found in bone marrow, two distinct adult stem cell types were recognized: (1) hemopoietic (HSC), which differentiated into the white blood cell population; and (2) mesenchymal (MSC), the progenitors of bone, cartilage, fat, and muscle.14,15 Endothelial precursor cells (EPCs), also known as angioblasts, are thought to derive from hemoblasts, a common ancestor for HSCs. MSCs and EPCs are now known to be present not only in bone marrow but also in fat tissue associated with the microvasculature,16 where they are known as adipose-derived stem cells (ASCs). Skin, muscle, cord blood, and amnion are also sources of MSCs. Tissue-specific stem cells have now been isolated from almost every organ as well as mesenchyme and they include cardiac, liver, lung, kidney, brain, breast, and others.
On activation, stem cells divide into a daughter cell committed to a particular lineage pathway given the appropriate differentiation stimulus and another which retains the stem properties. Most of our understanding of the properties of these cells comes from studies of bone marrow MSCs, but because of their ease of harvest by liposuction and their abundance, ASCs are now the preferred source. For the most part, they have the same properties but are more abundant, more easily cultured, grow more rapidly, and can be cultured for longer periods than bone marrow stem cells before senescence. A 100-mL sample of lipoaspirate can yield as many as 2 × 108 ASCs (estimated to range from 1/100 to 1/1500 cells).17 ASCs from different subcutaneous sites display differences in their density, differentiation potential, and adipose function.18–20 Their potential also varies with age.
Lipoaspirate is washed to lyse red blood cells, collagenase-digested, filtered, and centrifuged to produce a pellet of cells called stromovascular fraction (SVF). This fraction contains ASCs which can be isolated by their adherence to tissue culture plates. Other cells in SVF include EPCs, pericytes, fibroblasts, endothelial cells, anti-inflammatory M2 cells, and hemopoietic cells. Isolation of ASCs using surface markers has been elusive and there is no specific marker currently that specifies these cells. With progressive culturing of ASCs, the hemopoietic markers (CD34) are lost along with their angiogenic potential. They do not express markers of differentiated vascular origin (CD31 or hemopoietic CD45, nor human leukocyte antigen (HLA)-major histocompatibility complex (MHC) class II origin) and cannot be compared with primary isolated SVF cells. They regularly express CD29, CD44, CD90, and CD146. Rodeheffer et al. reported that CD24 was a key marker of ASCs.21 For tissue-engineering and fat injection purposes, it is clear that obsessive attempts to isolate the “pure ASC” may be counterproductive as the other cells of the SVF, especially the EPCs, may also contribute to the apparent functional effects of stem cell application.
For tissue-engineering purposes the ideal would be to have a stem cell that could be predifferentiated in vitro and then delivered in vivo where it would expand and become a particular tissue type. It is becoming clear that many of the initial claims for stem cell survival, differentiation, and their replacement of damaged tissue could not be substantiated. More commonly, increased angiogenesis or other beneficial anti-inflammatory paracrine effects are observed consistent with their known growth factor and cytokine capacities, including SDF-1, which allows them to home in to injured tissue.22–26
MSCs do not express MHC class II markers and several studies suggest they are relatively immune-privileged and may be used as allografts. This is a potentially attractive concept where cells could be sourced from stem cell banks for tissue engineering.27,28
Some researchers have persisted with ES cell research based on their allure of totipotency, their infinite capacity for proliferation, and the potential to study diseases at a genetic level. Successful differentiation protocols have been found to induce ES cells along specific lineage pathways from all germ layers towards many specific tissues and organs, including adipocytes.29 These cells are probably immunogenic and ethical issues will persist. There are many regulatory and organizational issues which will make pathways into the clinic difficult for elective procedures such as tissue engineering, especially proving that they will not form cancer.
Takahashi et al.30 described a method of converting somatic fully differentiated skin cells, mostly fibroblasts, to an embryonic-like state using four transcription factors. Unlike ES cells, they are derived from the patient and present no issues of allogenicity or ethics. They can be induced back through all germ layers to differentiated tissue types, potentially for repair of the donor. They are termed induced pluripotent stem (iPS) cells and they offer an unlimited supply of cells of specific donor identity. Disease models based on these adult-induced cells will also become powerful tools for investigations into genetically based diseases. Rather than using viral transfection to upregulate the induction genes, the proteins they produce can now be used for induction, eliminating issues of viral contamination and reducing the risks of cancer formation. Mice have been cloned from their own iPS cells by implantation into an enucleated ovum. These animals have reproduced and no cancers have been detected.31
Biochemical signaling
Metalloproteins, growth factors, and chemokines play vital roles locally and systemically in repair and tissue development, especially via macrophages. Inflammatory cytokines and local angiogenic growth factors stimulate endothelial cell migration, proliferation, and mitogenesis. Systemically, growth factor cytokines signal distant precursor cells, including EPCs.32,33 Embryogenesis is critically dependent on growth factor cascades released sequentially according to a genetic code and time clock. Gradient concentrations and specific time release of growth factors can theoretically be programmed into materials by incorporating them between layers with a controlled degradation profile to be released according to an orchestrated script but it is a tall order to imagine that anything but the simplest of growth factor combinations could be incorporated into bioengineered materials, so-called “smart surfaces,” so as to instruct cell behavior in vivo.
Biomechanical signaling and 2D and 3D culture
Cells change behavior in response to both their chemical and physical environments. When cultured on two-dimensional (2D) tissue culture plastic, the equivalent of cells being firmly stretched in clinical situations, their surface receptors sense the rigidity and adhere. They then move, migrate, and proliferate until they reach confluence. The same cells when placed in a soft 3D culture gel environment sense a modulus that encourages the cells to cluster together into spheroids (Fig. 19.3). Integrin-mediated adhesion between cells and the ECM modulates critical cellular events such as gene expression, embryonic development, cell movement, and differentiation. When the cells pull on the soft matrix they pull it into a ball, stop dividing, and many apoptose. If they are stem cells the environment promotes differentiation.34–36 By manipulating the ECM modulus cell behavior can be changed accordingly. Physiologically stiff substrates signal stimulation of tyrosine kinase or inhibition of tyrosine phosphatase which stabilizes focal adhesions. The cell measures the flexibility of its substrate cytoskeleton myosin push/pull on the focal adhesion. The response then leads to changes in tyrosine phosphorylation to signal transduction to the nucleus. The mechanical properties of the cell’s surrounding substrate environment could be modulated by synthesis/degradation of ECM proteins, movement of cells, or fluid shear from blood flow. These events continually occur during embryonic development and wound healing.37
Thus responses to culture in 3D can differ significantly from those on 2D surfaces.38–43 Culture on traditional hard 2D surfaces such as tissue culture plastic does not replicate the 3D architecture or physical cues of the natural cellular microenvironment in the ECM and it has been shown that a 3D culture environment is important to physiological function of cells in vitro. Chondrocytes, for example, change morphology and lose their chondrogenic capacity in 2D monolayer culture but can maintain these features in 3D culture.44
Distraction osteogenesis exploits these phenomena. Periods of stretch promote proliferation and migration while relaxation incites cells to cluster together and terminally differentiate into bone. GTR similarly works by presenting the cells with a hard surface which stimulates them to divide and to migrate. Stem cell mechanobiology is attracting increased attention as understanding develops of the influence of substrate mechanics and architecture on differentiation.45 Cells may be cultured in 3D on biomaterial supports such as porous scaffolds, hydrogels, or microspheres under conditions designed for the desired cell attachment, migration, proliferation, and differentiation.46 The design of such biomaterial constructs is discussed further later. Bioreactors which magnify cell proliferation or differentiation in culture are based on the same principles of manipulating mechanical forces, such as fluid flow and substrate flexibility.
Co-culture
Tissues cultured ex vivo generally are of one cell type and following implantation into an animal rely on recruitment of supporting cellular structures and matrix to evolve into a stable, functional tissue. Co-culturing cells with other types prior to implantation can facilitate their survival and function, such as endothelial cells for vasculature47 or MSCs for paracrine effects, or for their ability to incorporate into developing tissues in vivo such as blood vessels.16 In vitro co-culturing beating cardiomyocytes with ASCs to initiate differentiation into cardiac lineage is a further application.48
Bioreactors
Cell culture in 3D constructs presents challenges in delivery and removal of nutrients and waste products in the absence of a blood supply.49 The delivery of oxygen is challenging due to its relatively low solubility in cell culture media and the time required for diffusion to occur into human-scale 3D constructs. Delivery of macromolecules such as growth factors also presents challenges due to their large size and thus relatively slow diffusion. In addition, mechanical forces and hydrodynamic conditions can significantly affect cellular processes and tissue development. Therefore, bioreactors may be used to provide fluid flow around or through the construct to enhance mass transport and suit the hydrodynamic requirements of the tissue.
It is challenging to obtain uniform conditions throughout a bioreactor due to the difficulty of seeding cells uniformly, mass transport limitations, and variations in fluid shear rates, and this can lead to nonuniform tissue development. Bioreactors which apply additional mechanical forces on the developing tissue construct are also used, particularly for tissues which experience significant forces in vivo, like tendons and ligaments. The significant effects of physical forces were demonstrated in engineered cartilage constructs grown in rotating bioreactors in microgravity in comparison to controls grown on Earth (Fig. 19.4).50 The constructs grown in microgravity for 4 out of the 7 months’ culture time showed markedly lower aggregate modulus and glycosaminoglycan content than constructs grown for 7 months on Earth, which showed comparable values to natural cartilage.
The challenges and approaches developed for cell culture for tissue engineering of a range of specific tissue types have been reviewed in the text edited by Vunjak-Novakovic and Freshney.51
Applications for stem cells and tissue engineering
Specific tissues grown using stem cells with traditional tissue-engineering techniques are discussed later. Initial reports of injecting MSCs into tissues for various indications, including cardiac, peripheral vascular disease, and irradiation injury in humans as well as a large experience in the horse-racing industry, boasted survival, differentiation, and function. Few studies were controlled and tracking of cells was absent or difficult to assess. With the exception of brain, almost no cases could confirm that the MSCs survived in significant numbers or that these survivors differentiated into the injured tissue type. Most studies now concede that the paracrine-growth factor-hormonal-cytokine immune-modulatory effects probably account for the benefits seen with these stem cells. Ischemia increases homing of these cells to the injured site where MSCs release high levels of vascular endothelial growth factor (VEGF) to modulate the repair of capillaries. MSCs injected intravenously in cardiac infarct models do not implant in the heart nor become heart tissue but lodge in the lung, where they are activated to secrete the anti-inflammatory protein TSG-6,23 and it is probably the anti-inflammatory factor that induces the beneficial effects. Partially purified ASCs which still retain CD34 and CD90 markers grown into spheroid clusters in gel culture differentiate into endothelial cells and capillary structures in methyl cellulose and these produce high levels of VEGF. They also rapidly differentiate into adipocytes in adipogenic media, making them potentially suitable for adipose tissue engineering.52 Nonexpanded SVF has shown efficacy after injection into Crohn’s tissue and in equine studies due to its anti-inflammatory effects.53 More recently, MSCs have been used as intravenous infusions for the treatment of multiple sclerosis, with promising results. It is felt that the response was anti-inflammatory in nature due to the release of high numbers of M2 anti-inflammatory macrophages.54 Other growth factors released from ASCs include macrophage colony-stimulating factor, GMCSF, VEGF, antiapoptotic factors, hepatocyte growth factor, and transforming growth factor-β (TGF-β1). In hypoxia, the VEGF levels are significantly increased, leading to angiogenesis and decreased apoptosis.
Matrix
Biomaterials
An increasing variety of synthetic and naturally derived biomaterials have been considered for application in tissue engineering. Early tissue-engineering attempts tended to utilize materials which had already been used in other clinical applications, such as the polymers used in degradable sutures. Natural materials such as porous coral and hydrogels like alginate or collagen were also trialed. More recently, numerous alternatives have been formulated for particular tissue targets as researchers aim to develop multifunctional biomimetic biomaterials with superior performance characteristics to suit the specific demands of tissue engineering, which can differ significantly from those of other biomaterial applications. Newly developed materials require extensive testing in vitro and in vivo before clinical acceptance, with very high costs and relatively low rates of translation from the laboratory to the clinic to date.55 Despite this, a range of such materials are in this testing pipeline.
Criteria for biomaterials to be suitable for tissue engineering applications include:
• biocompatibility, i.e., eliciting a suitable host response in the specific application
• suitable mechanical properties for target tissue and implantation site
• biodegradability over desired timeframe with suitable mechanical properties and nonharmful degradation products
• not containing harmful impurities
• a host response in vivo that does not prevent desired tissue development; i.e., inflammation and foreign-body response are not excessive
• ability to be fabricated into desired structures
• ability to be sterilized without harmful changes to their chemical or physical properties
• adequate stability and shelf-life
• ability to promote desired cellular responses, e.g., proliferation, differentiation, gene expression.
Biomaterials currently available for use in tissue-engineering approaches do not generally ideally satisfy all of these criteria and development of better biomaterials for tissue engineering is an area of active research worldwide. Whilst it may be feasible to produce complex biomimetic materials to influence cell fate ex vivo, simplicity is also desirable to facilitate regulatory approval and translation of new materials into clinical application.56 Selection of biomaterials will depend on the specific requirements of the tissue being targeted.
Degradation of biomaterials may result in relatively sudden changes in their properties, such as their mechanical strength before the biomaterial is completely removed. The rate of degradation can vary widely so the mechanical integrity may be retained for as little as days or weeks, or as long as a year or more. The rate of degradation and loss of integrity will depend on the type of biomaterial, the site of implantation, properties of the biomaterial construct such as surface area to volume ratio, size, and surface chemistry. Figure 19.5 shows examples of the loss of mechanical strength of biodegradable polymers tested in vitro. As well as the loss of physical integrity, rapid degradation may lead to excessive concentrations of the degradation products of a biomaterial which can cause adverse tissue reactions. This is well known for the polylactides and polyglycolides which can undergo autocatalytic degradation with decreasing pH inside a thick-walled construct and “acid burst” leading to reduced local pH and tissue necrosis. For this reason, it is important to consider degradation rates and mechanisms in designing the composition and architecture of tissue-engineering constructs using biomaterials.
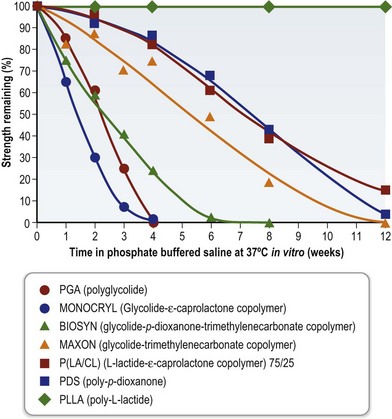
Fig. 19.5 Changes in tensile strength of biodegradable polymers during degradation in vitro.
(Reproduced from Suzuki M, Ikada Y. Biodegradable polymers in medicine. In: Reis RL, Román JS (eds) Biodegradable systems in tissue engineering and regenerative medicine. Boca Raton: CRC Press, 2005.)
Natural biomaterials
Natural biomaterials may have the benefits of being chemically similar or identical to molecules in the body, readily degraded in vivo, and able to interact with cells on a molecular level. However, they can be difficult to obtain and purify, vary in properties between batches, may be difficult to sterilize, alter their properties during storage, and they may elicit significant immunogenic responses. Naturally derived biomaterials investigated for tissue engineering include proteins (e.g., collagen, gelatin, silk), polysaccharides (e.g., chitosan, hyaluronic acid), polynucleotides and extracts of ECM components.57 These materials may be modified to tailor their properties, such as via chemical cross-linking to slow degradation and increase mechanical strength.
Synthetic biomaterials
Numerous synthetic polymers are used in medicine and some of these are biodegradable (or bioresorbable) in vivo. Figure 19.6 shows examples of the chemical structures of biodegradable polymers that could be used in tissue engineering. Among these, the polyesters poly(glycolic acid), poly(lactic acid) and poly(ε-caprolactone) (PCL) and their copolymers such as poly(lactide-co-glycolide) (PLGA) have been widely used in tissue-engineering approaches.58,59 The mechanical strength and degradation rate of these polymers can be tuned over a wide range by changing the polymer properties (molecular weight, composition, crystallinity).60 As can be seen from the chemical structures, many biodegradable synthetic polymers are quite hydrophobic, unlike the ECM. Exposure of such surfaces in vivo can lead to nonspecific adsorption of proteins of mixed orientation and states of denaturation, potentially resulting in a foreign-body reaction (FBR) to the material.61
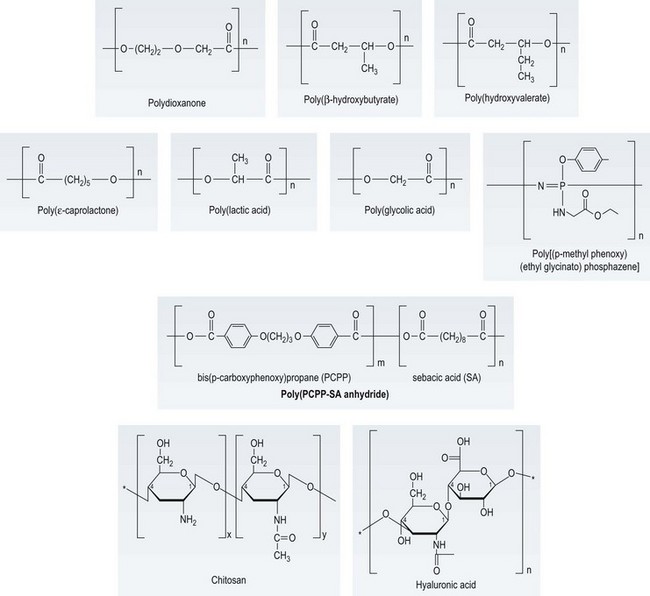
Fig. 19.6 Chemical structures of selected biodegradable polymers of potential interest in tissue engineering.
(Reproduced from Kohn J, Abramson S, Langer R. Bioresorbable and Bioerodible Materials. In: Ratner BD, Hoffman AS, Schoen FJ, et al. (eds) Biomaterials Science. Amsterdam: Elsevier, 2004, pp. 115–217. Chitosan reproduced from Guibal E, Vincent T, Blondet FP, Biopolymers as supports for heterogeneous catalysis: Focus on chitosan, a promising aminopolysaccharide, in Ion Exchange and Solvent Extraction: A Series of Advances A. SenGupta, Ed. 2007, Taylor & Francis: Boca Raton. 151–292. Hyaluronic acid reproduced from Yannas, IV, Natural Materials, in Biomaterials Science – An Introduction to Materials in Medicine, B.D. Ratner, et al., Eds. 2004, Elsevier: Amsterdam.)
Hydrogels
Hydrogels are water-swollen cross-linked polymer networks which can absorb up to thousands of times their dry weight of water, which gives them the advantage of being more like most natural tissues and allowing mass transport to and from cells.49,62 They also allow relatively easy incorporation of cell-binding ligands or ECM molecules to facilitate cell interactions. They may be formed by physical (hydrogen bonds, ionic interactions, molecular entanglements, hydrophobic interactions) or chemical interactions (covalent bonds) and this will affect their stability and degradation rates. The mechanical strength of hydrogels is limited and their degradation tends to be faster than for other biomaterials. Hydrogels may be naturally derived (e.g., collagen, gelatin, hyaluronic acid, alginate) or synthetic (e.g., poly(ethylene glycol) (PEG)-based polymers).63
Gelation or changes in molecular conformation may be caused by environmental changes, resulting in so-called smart polymers, as shown in Figure 19.7. These types of change can be used to encapsulate and release payloads of cells or drugs, to form gels upon injection in vivo, or for cell sheet engineering.61,64,65
Research efforts to improve biomaterials for tissue engineering include efforts to create cell-responsive materials, which degrade in response to cellular processes (e.g., secretion of matrix metalloproteinase) during tissue development such that the biomaterial degradation rate adapts to the tissue growth rate.66 Another key challenge is the FBR seen in vivo with almost all synthetic biomaterials used clinically, be they polymers, ceramics, or hydrogels.
Approaches to reduce the FBR to biomaterials in vivo may use physical properties, such as by creating spatial features which lead to reduced fibrosis (e.g., porous polymers with pores of around 30 µm67 or small-diameter fibers68). Surface chemical properties can also be used to reduce the FBR to biomaterials, such as via attachment of oriented cell adhesion ligands (e.g., oriented osteopontin on a polymer surface69), or by modifications to minimize nonspecific adsorption of host proteins, which can be denatured and lead to an immune reaction (e.g., by layer-by-layer assembly of polyelectrolytes ending with a nonadhesive outer layer,70 or attachment of PEG71). However, many strategies which show promise in vitro suffer from enzymatic attack and changes to the biomaterial surface in vivo during the acute inflammatory stages of the wound-healing response, and so their in vivo performance may be disappointing.72
Tissue-engineering construct fabrication
Biomaterial constructs for tissue engineering can be fabricated into structures such as porous scaffolds and hydrogels, meshes, or microspheres using a variety of techniques, such as the solid free-form fabrication and rapid prototyping methods illustrated in Figure 19.8. In addition to these methods, polymer phase separation, particle or foam templating, and electrospinning have been widely investigated to produce 3D constructs for tissue engineering.39,73–75 The techniques for tissue-engineering scaffold fabrication have been recently reviewed.55,76–78
• biomaterial composition (chemical composition, molecular weight, polydispersity)
• internal 3D porous architecture
• surface chemistry and roughness
• degradation properties and products
• mechanical properties (initially and during degradation)
• effects on cellular processes (adhesion, migration, proliferation, and differentiation)
• ability to be fabricated reproducibly and economically
• ability to be sterilized without unacceptable property changes.
The interaction of cells with a biomaterial surface alters depending on whether the surface has binding sites of suitable orientation and conformation to enable attachment of the cells, and the density of such sites. This in turn can affect cell morphology and migration rates, as shown in Figure 19.9.
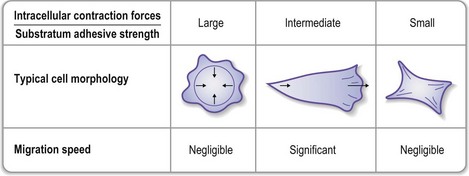
Fig. 19.9 Effects of cell–substrate interactions on cell migration speed.
(Reproduced from Palsson BO, Bhatia SN. Tissue Engineering. Upper Saddle River, NJ: Pearson Education, 2004.)
Scaffolds of various internal architectures can be produced, as shown in Figure 19.10. The porosity must be interconnected and of sufficient size for molecular transport, cell migration, and vascularization. The size required may be larger than the minimum size required to fit cells or blood capillaries. Cao et al. found that pores of at least 300 µm were needed for tissue survival in PLGA scaffolds where a significant FBR occurred inside the scaffolds.79 Scaffolds may have up to 90% porosity or more and the extent and architecture of the porosity can be used to control the scaffold strength, as shown in Figure 19.11. Ideally the mechanical strength of tissue-engineering constructs would be designed to match that of the tissue targeted to favor the desired cellular behavior, as discussed above, and avoid stress shielding, which can lead to osteolysis in the case of bone implants.80
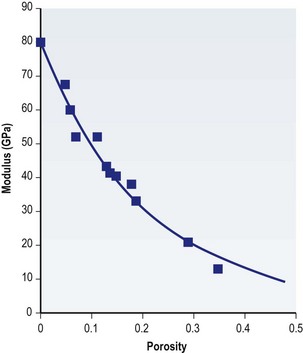
Fig. 19.11 Ceramic scaffold mechanical properties as a function of porosity.
(From Boccaccini AR. Ceramics. In: Hench JRJ (ed.) Biomaterials, artificial organs and tissue engineering. Cambridge, UK: Woodhead, 2005.)
The mechanical properties of tissues and their components vary widely (Table 19.1 and Fig. 19.12A) and so the physical microenvironment of particular cells in vivo also varies significantly. Engler et al.36,45 have shown that MSC lineage specification can be altered in vitro when cells are cultured on substrates of different stiffness (Fig. 19.12
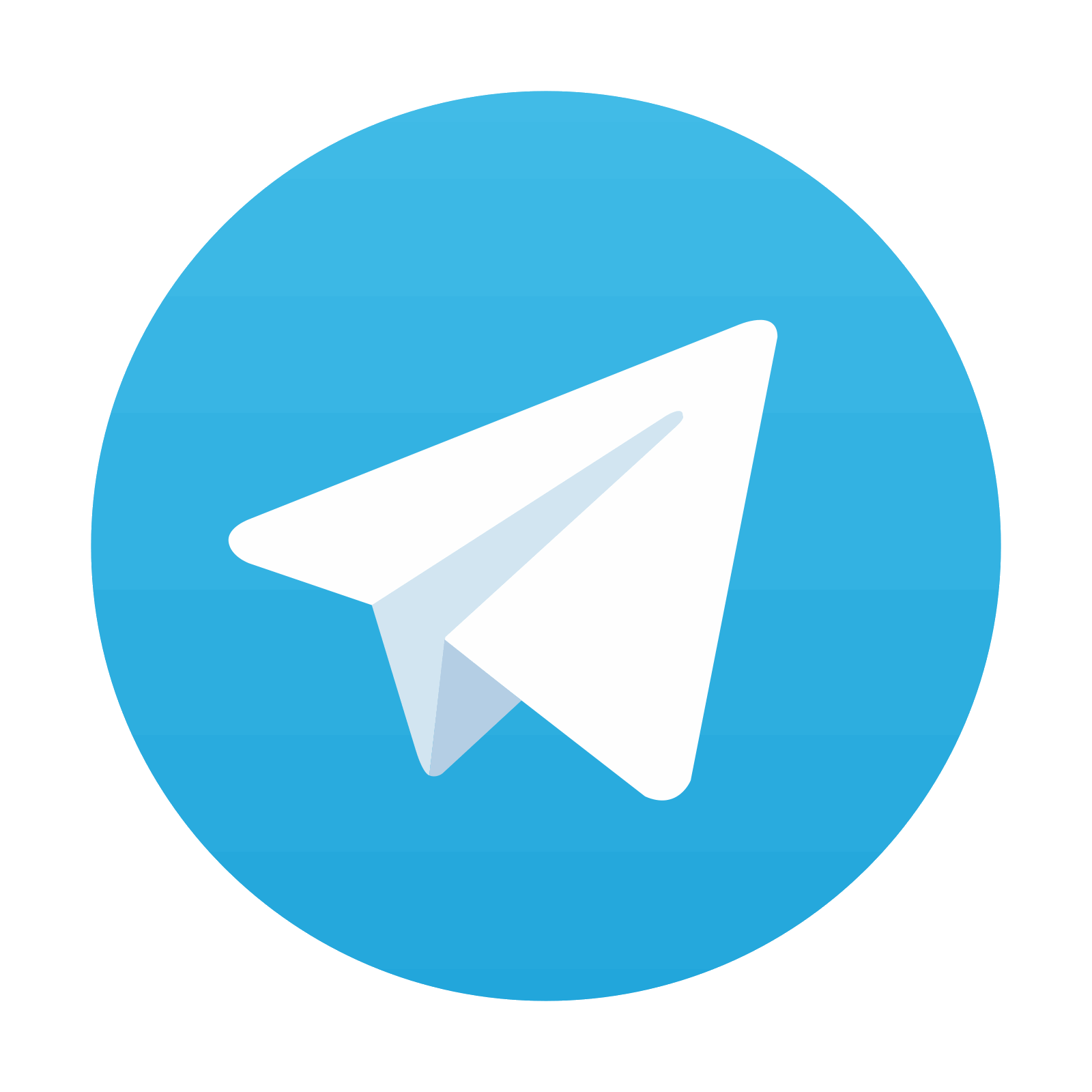
Stay updated, free articles. Join our Telegram channel
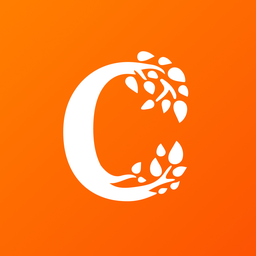
Full access? Get Clinical Tree
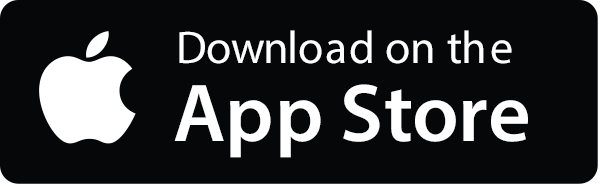
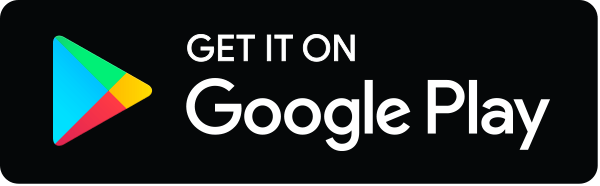