Radiation Principles
Radiotherapy is the medical use of ionizing radiation, generally as part of cancer treatment to control or kill malignant cells. Ionizing radiation is composed of particles that individually carry enough kinetic energy to liberate an electron from an atom or molecule. Directly ionizing radiation consists of alpha and beta particles. Indirectly ionizing radiation includes photon radiation which may be gamma radiation if produced by radioactive decay or X-rays if produced outside the nucleus.
Alpha Particle Radiation
An alpha particle is identical to a helium nucleus, containing 2 protons and 2 neutrons. Alpha particles are generally produced by a process of alpha decay. If ingested, inhaled or absorbed into the bloodstream, they can cause severe damage. Radium-226, radon-222, and polonium-210 are alpha emitters. Most alpha emitters occur naturally in the environment and are found in various amounts in almost all rocks, soils, and water. This form of radiation is rarely used for the treatment of cancer.
Beta Particle Radiation
Beta particles are high-energy, high-speed electrons or positrons emitted by certain types of radioactive nuclei. Cobalt-60, strontium-90 and iodine-131 are examples of some of the beta emitters. Iodine-131 is used in the treatment of benign and malignant thyroid disease. Strontium-90 is used in the treatment of metastatic bone cancer and has also been used for treating ocular melanoma. Beta particles are also a source of positrons used in positron emission tomography (PET).
Gamma Radiation
Gamma rays are produced by nuclear gamma decay and are emitted from a radioactive source, often following the emission of a beta particle. Gamma rays are less ionizing but more penetrating than alpha and beta rays and can be stopped by a few centimeters of lead. The commonly used gamma emitters are caesium-137, cobalt-60, and technetium-99m. Technetium is widely used for diagnostic studies, whereas caesium and cobalt are used in the treatment of cancer.
X-Rays
X-rays, like gamma rays, are part of the electromagnetic spectrum. They are emitted by electrons. X-rays of varying energies have many uses but are best known for their medical application. Low-energy X-rays (∼10 keV) are used in diagnostic radiography to take images of part of a patient’s body (e.g., chest or abdomen). The differential absorption of the X-rays by bone, air, and soft tissue generates a clear radiograph. High-energy X-rays (photons) are able to ionize atoms and disrupt molecular bonds, thereby causing potential damage to the tissues they pass through. The ionizing capability of X-rays is utilized in radiotherapy, which uses photons to kill malignant cells in the treatment of cancer. In radiotherapy the photons are generated by a machine called a linear accelerator (linac). The linac generates electrons and then speeds them up to almost the speed of light using electrical fields. As the electron is accelerated its kinetic energy is increased until such time as it collides with the target and the energy is released as a photon (Bremsstrahlung radiation).
Mode of Action in Malignancies
When radiation interacts with cells, ionization of DNA molecules takes place causing tumor cell death. The biological effect of ionizations is controlled by a number of factors, such as the number of ionizations that can cause DNA damage, free radical scavenging processes, and the degree of cellular repair. There are two mechanisms by which radiation causes cell damage: direct and indirect.
Indirect Effect
Approximately 60% of the human body is made of water; therefore the majority of ionizations generated by irradiation arise in the water molecules. When radiation interacts with water it breaks the bonds that hold the water molecule together, producing a positively charged hydrogen ion (H ++ ) and an uncharged hydroxyl radical (OH − ). The OH radical is often called a free radical as it has an unpaired electron and is highly reactive, causing cell damage. These free radicals may undergo further reactions to produce hydrogen peroxide, which also contributes to the destruction of the cells. Oxygen plays a key role in this process of radiolysis. Approximately two-thirds of the biological damage caused by X-rays or electrons is by indirect effect.
Direct Effect
If radiation causes ionization of the atoms that are part of the DNA molecule, or some other cellular component essential for the survival of the cell, then the cell may be destroyed directly by interference with its life-sustaining structure. This is referred to as the direct effect of radiation.
Biological Effect on the Cell Cycle
Cell cycle progression is vital for the growth and survival of a cell or organism ( Fig. 12.1 ). The normal cell proliferation cycle goes through two main phases: mitosis (M phase) and DNA synthesis (S phase), which are separated by the G 1 (before cell division) and G 2 (after cell division) phases. G 0 is the resting phase before cell division begins. The average length of a cell cycle is 16 h; this is shorter for cancer cells. Cell cycle arrest normally occurs in response to events that threaten the integrity of the DNA. Cells are most sensitive to radiation in the M and G 2 phases and most resistant in the S phase of the cell cycle. After radiotherapy is delivered, cells may be in different phases of the cell cycle. Fractionating radiotherapy may allow resistant cells time to enter the sensitive phase of the cycle and be more amenable to cell kill. Following radiotherapy, DNA damage can also cause a progressive delay in G 1 , S, and G 2 phases of the cell cycle. This delay gives the cells time to repair the damage.

Radiation Injury
Radiation kills actively dividing cancer cells efficiently; damage to cancer cells may occur immediately but may take months to take full effect. Along with the cancer cells, some rapidly dividing normal cells (skin, intestines, hair, and bone marrow) are also damaged in the process, leading to unwanted acute effects. Slowly dividing normal cells (nerves, brain, bone, vessels) exhibit radiation-induced damage later.
Radiation-related injury to the cells is divided into three main categories:
- •
Lethal damage is a complex, irreparable and irreversible mechanism leading to cell death. The cell is unable to repair the damage and gives rise to a linear component of cell killing.
- •
Sublethal damage following ionizing radiation can be repaired within hours of the injury taking place and would not lead to cell death, unless further radiation exposure was inflicted on the cells. Cellular repair mechanisms can protect them from further injury, which is important for normal tissues, as they are able to repair themselves between fractions of radiotherapy. The approximate time needed for half the sublethal damage to be repaired (half-life) is different for different tissues. The recovery time is approximately 1 h and is thought to be complete by about 4 h in mammalian cells.
- •
Potentially lethal damage will inevitably kill the cells unless manipulated by repair-facilitated cell cycle arrest. Normal cells can readily be repaired in the S phase but not in the M phase. Tumor cells have defective cell cycle controls and therefore do not undergo cell cycle arrest required for repair, in contrast to the normal cells. A minimum of 6 h are required between fractions of radiotherapy to provide sufficient time for these repair mechanisms to work.
Modes of Delivery
Radiotherapy delivered by a machine is often referred to as external beam radiotherapy. It may also be delivered by placing a radioactive source inside the body, which is referred to as brachytherapy.
External Beam Radiotherapy
This is the commonest form of radiotherapy used for the treatment of cancer. Different machines are used for the delivery of varying energies.
The superficial machine produces X-rays that range from 50 to 150 kV (kilovolts) and is used for treating superficial skin lesions. It deposits most of its energy at the surface of the skin and 90% of the dose at a depth of approximately 5 mm from the surface of the skin.
The orthovoltage machine generates X-rays ranging from 150 to 500 kV energy, depositing maximum energy near the surface of the skin and 90% of the dose at a depth of approximately 2 cm from the skin surface. It is also used for treating superficial tissues, skin, and bone lesions.
The megavoltage machine produces X-rays of higher energies, usually 1 MV (megavolt) and above. A linear accelerator (linac) is commonly used for the delivery of these high-energy beams. The maximum dose of radiotherapy can be delivered to various depths depending on the energy of the beam used, aiding treatment of deep-seated tumors. Most linacs can also be adapted to treat superficial lesions, such as in cases of skin cancer. This treatment uses electrons instead of high-energy photons. Further technical advances in the design of the linac has permitted the use of intensity-modulated radiotherapy (IMRT); volumetric modulated arc therapy (VMAT); stereotactic radiotherapy (SBRT); image-guided radiotherapy (IGRT) and magnetic resonance imaging (MRI)-guided radiotherapy. All of these techniques allow shaping and modulation of the radiation beam to reduce radiation dose to normal tissues whilst intensifying dose to target.
Particle therapy using high-energy protons allows high doses of radiation to be delivered to a specific depth range with less entry and minimal exit dose. For this reason, proton beam therapy (PBT) has historically been used to target tumors that lie in close proximity to a critical organ at risk (OAR) that cannot be adequately spared using photons (e.g., paraspinal and skull base tumors). However, there is widespread interest in using protons in a variety of tumor sites where a significant reduction in the radiation dose delivered to normal tissues may be achievable.
Brachytherapy
Brachytherapy is a mode of delivering radiotherapy by using sealed radioactive sources that can be placed either inside a cavity containing the tumor (intracavitary) or directly in the tumor itself (interstitial). Other forms of brachytherapy include intraluminal, intraoperative, intravascular, and surface brachytherapy.
The main radioactive sources used for brachytherapy are iridium, caesium, and iodine.
The advantage of brachytherapy over external beam radiotherapy is the ability to deliver higher doses of radiation to the tumor and lower doses to the surrounding normal tissues. There tends to be a rapid fall-off in dose as the distance increases from the radioactive source, which restricts its use to the treatment of small and well-localized tumors.
Unsealed radioactive sources are also used for treatment and can be delivered either orally or intravenously. However, following treatment, limited-period precautions are necessary to avoid radiation exposure to individuals in close contact. Radioactive iodine-131 used in the treatment of thyroid cancer is an example of this form of treatment.
Fractionation
Curative treatment of cancer requires higher doses of radiation in order to produce sufficient cell killing. The total dose is delivered in small daily fractions over a period of time to minimize toxicity by allowing sufficient time for normal tissue recovery while inducing tumor cell kill. The efficacy of fractionation is based on the four Rs of radiobiology: repair of sublethal damage, redistribution of cells within the cell cycle, repopulation, and reoxygenation.
By splitting radiation into small daily fractions, normal cells with efficient repair mechanisms are able to repair sublethal damage in contrast to cancer cells, which have suppressed repair pathways. Both normal and malignant cells repopulate between fractions. Prolonging total treatment time over several weeks allows normal cell repopulation; however, some rapidly dividing tumors such as squamous cell carcinomas regenerate faster than the normal cells and exhibit accelerated repopulation. Fractionation increases damage to the tumor by allowing reoxygenation of the tumor environment and redistribution of the cells into radiosensitive phases of the cell cycle.
Conventional (standard) fractionation is the delivery of 1.8–2 Gy per day, given as five daily treatments a week over several weeks. Other fractionating regimens used are as follows:
- •
Hyperfractionation: The same total dose is delivered over the same time as in standard fractionation but more than one fraction is given per day at a lower dose per fraction. This reduces the late effects of radiotherapy.
- •
Accelerated treatment : The total dose is delivered over a shorter period of time compared with standard fractionation in order to overcome accelerated tumor repopulation.
- •
Continuous hyperfractionated accelerated radiation therapy (CHART) combines the above two approaches and is used in the treatment of rapidly proliferating tumors (e.g., lung cancer and some head and neck malignancies). It consists of three smaller fractions of radiotherapy per day, separated by 6 hours to allow recovery of normal tissues.
- •
Hypofractionation: Radiotherapy is delivered in a smaller number of fractions with a higher dose per fraction. There is potentially a higher risk of developing late effects in normal tissues with this regimen. It is used in the treatment of slow-growing tumors, which are generally resistant to standard fractionation schedules, and also in the palliative setting where development of late effects is less relevant.
Dose and Dose Rate
Dose is the general term used to determine the quantity of radiation. The energy imparted by ionizing radiation to matter at a specific point is defined as absorbed dose. The SI unit of absorbed dose is joule per kilogram (J/kg) also known as gray (Gy).
Dose rate is defined as the radiation dose delivered per unit time and measured in grays per hour. Radiation response decreases with decreasing dose rate and this is referred to as dose rate effect. The typical dose rate used in standard radiotherapy is 1 Gy/min.
Effects of Oxygen and Radiotherapy
Malignant cells are most sensitive to radiotherapy in the presence of oxygen. Hypoxic cells are radioresistant and are usually present in the core of the tumor, whereas the cells on the surface of the tumor have an abundant blood supply and are well oxygenated. As the tumor is exposed to radiotherapy, the outer, well-oxygenated layers of the tumor are rapidly destroyed and the hypoxic inner layers become oxygenated and thus radiosensitive between treatment fractions.
Treatment Parameters
Total Dose
Total dose refers to the total amount of ionizing radiation absorbed by the patient (expressed in Gy). Every tissue in the body has a tolerance dose beyond which the organ would be at risk of serious complications. Various dose/fractionation schedules can make it difficult to assess the total dose delivered to a particular organ. Therefore, a formula is applied to compare altered fractionation doses to standard 2 Gy per fraction doses to know the exact total dose delivered to an organ. This is referred to as a biologically equivalent dose.
Dose Fraction Size
Fraction size is a significant factor influencing the development of acute and late effects of radiotherapy. Large fraction sizes increase the severity of late normal tissue damage.
Volume Treated
The severity of acute and late normal tissue injury from radiotherapy depends on the volume of tissue irradiated. Tissues with a parallel organization, such as the lung and kidney, have a high functional reserve and exhibit a prominent volume effect. This means that the damage caused would depend on the dose distribution to the whole organ rather than to a small area. On the other hand, serial organs such as the spinal cord and gastrointestinal tract have little functional reserve and it is vital that radiation dose does not exceed tolerance at any point.
Time Elapsed
If the overall treatment time is increased, a greater amount of repopulation of the irradiated normal and tumor cells is permitted. This has an adverse effect on local tumor control, especially in rapidly proliferating tumors.
Radiation Effects
Acute Toxicity
The acute effects of radiotherapy are generally defined as those toxicities occurring up to 90 days from completion of treatment. Acute effects usually manifest in rapidly dividing cells in the area being treated and are thought to be due to depletion of stem cells. Common sites exhibiting early radiation effects are the skin (including hair follicles and sweat glands), mucous membranes of the gastrointestinal tract, and hematopoietic tissue.
If photon radiation is used it can take some weeks into the treatment course before erythema becomes evident, as initial dose is deposited in the deeper dermis. If a higher skin dose is required (e.g., when tumor involves the skin or in the treatment of a primary skin cancer), a tissue-equivalent bolus material may be applied over the treated field to increase the surface dose. Adjustments in radiation technique to use electrons or orthovoltage (less penetrating) radiation also increase the skin dose and result in the development of skin erythema within the first week of a fractionated course of radiotherapy.
The rates of onset of damage and recovery from it depend upon the turnover rate of epithelial cells. In a typical 6-week course of radical radiotherapy delivered with curative intent one would expect skin erythema and inflammation to develop at the treated site within the first 3 weeks. Epilation also occurs early and dry desquamation of the skin may follow as surface flaking of the stratum corneum. Towards the end of the course of radiotherapy moist desquamation of the skin is often seen, as exudate is released from the damaged skin cells. This is more commonly seen at the skin creases of the axillae and groins.
In radiotherapy to the head and neck, mucositis develops over the first few weeks, ranging from patchy isolated areas to confluent areas with ulceration. Taste disturbance is noted and salivary gland function is diminished. The latter results from acinar atrophy and chronic inflammation of the salivary glands.
Late Toxicity
The late effects of radiotherapy are generally the dose-limiting factor. High total dose, large fraction sizes, and large treatment volumes all contribute to a greater risk of late effects. The main mechanisms for the late effects of radiotherapy are believed to be damage to vascular and connective tissue and a reduction in the proliferative capacity of stem cells. Late effects tend to manifest in slowly proliferating tissues (e.g., lung, kidney, liver, heart, and nervous tissue).
Late normal tissue damage reflects a failure to regenerate functional tissue, whether through lack of stem cells or radiation-induced dysregulation of the normal healing process. This latter phenomenon is described as a result of recent research into radiobiology and molecular pathology of late radiotherapy effects. Cytokine cascades are activated early at the cell and tissue level after irradiation and remain active throughout the expression of late tissue damage. Skin atrophy, telangiectasia, necrosis, and fibrosis occur many months after radiotherapy. Skin atrophy is seen as shrinking and thinning of the skin in the irradiated field. Telangiectasia results from a failure in the regrowth of normal vasculature. It has been postulated that telangiectasia results from loss of smooth muscle cells in vessels, leading to a loss of capillary pressure control. Loss of endothelial cells as a result of radiotherapy may also contribute to its formation. Necrosis is generally seen in atrophic skin, often developing as a result of trauma, and reflecting the impaired reaction of the vasculature to tissue injury. Many late effects of radiotherapy can be attributed to fibrinogenesis and excessive extracellular matrix and collagen deposition. Increased knowledge of the relevant cytokines and their actions has provided molecular targets for potential therapeutic intervention.
Osteoradionecrosis
Osteoradionecrosis (ORN) is defined as an area of exposed bone in a field of radiation that has failed to show any evidence of healing for 6 months with the absence of recurrent or residual tumor. The incidence of ORN is believed to have declined considerably over time; reported incidence ranges from 5% to 7%.
The pathophysiology of ORN is described as a cumulative tissue damage induced by radiation. The ‘three Hs’ principle outlines how radiation results in a hypocellular, hypovascular, and hypoxic tissue environment. Tissue breakdown occurs as a result of cell death and collagen lysis exceeding cellular replication. The result is progression to a nonhealing wound.
A key event in the progression of ORN is the activation and dysregulation of fibroblastic activity that leads to atrophic tissue within a previously irradiated area. High-dose radiation leads to bone death via a combination of mechanisms described as progressive resorption of osteoclasts, periosteocyticlysis, extensive demineralization, and accelerated aging of bone.
Common sites for bone necrosis are the jaw (soft tissue tumors of the head and neck), ribs, and sternum (breast cancer), skull (brain tumors and soft tissue tumors of the scalp), and vertebral column (spinal cord tumors).
Advanced (stage III or IV) tumor/recurrent tumors, tumors invading bone, and tumors involving floor of mouth, tongue, and retromolar trigone have been shown to have a higher risk of ORN. Treatment-related factors contributing to the development of ORN include large treatment volumes, high radiation dose, and dose per fraction, with the highest incidence occurring with total radiation dose above 70 Gy. Modifiable risks include smoking, the use of bisphosphates, and poor dentition. Patients should have a thorough dental assessment and extraction of any teeth in poor condition prior to commencing a course of radiotherapy to the head and neck in order to optimize oral health, though ORN has been seen in edentulous patients also.
The presentation of ORN varies with site. In cases of ORN of the mandible patients may present with pain, swelling, and trismus. ORN of the temporal bone can present with severe otalgia and offensive otorrhea. Exposed bone and pathological fracture may also be seen. Imaging with plain radiographs may highlight areas of local decalcification or sclerosis. Computed tomography (CT) or MRI scanning is used to delineate the affected area prior to definitive surgery. A deep biopsy of the lesion to rule out recurrent malignancy is generally recommended.
Treatment of ORN involves initial measures such as control of any superadded infection, gentle irrigation of the soft tissues, nutritional support, and analgesia. There is conflicting evidence regarding the use of hyperbaric oxygen therapy (HBOT) in the management of ORN, with some studies showing increased likelihood of attaining mucosal cover with HBOT. There remains no national or international guidelines and practice is dictated by local policy.
Radiation Therapy and Wound Healing
The wound-healing capacity of irradiated skin may be severely impaired, with clinical implications for patients requiring treatment combining radiation and surgery. Approximately 60% of patients with solid malignant tumors receive radiotherapy as part of their treatment, either with curative or palliative intent. Radiotherapy is often used after surgery in malignant disease in cases of narrow or involved excision margins or to nodal basins post lymphadenectomy to reduce the risk of local disease relapse and improve overall survival. Radiotherapy may also be used before surgery to downstage a tumor prior to definitive resection (e.g., in preoperative radiotherapy for rectal cancer and extremity soft tissue sarcoma).
The process of wound healing is well described to occur in three phases: an inflammatory phase, which develops within days; a proliferative phase, which occurs over many days to weeks; and a remodeling/maturation phase, which extends from many weeks to years.
Unlike a surgical wound, however, fractionated radiotherapy results in repetitive injury to soft tissues. This results in an accumulation of inflammatory responses and dysregulation of the highly structured process of wound healing.
Cytokines known to be involved in the initial inflammatory response to tissue damage are transforming growth factor beta (TGF-β), vascular endothelial growth factor (VEGF), tumor necrosis factor alpha (TNF-α), interferon gamma (IFN-γ), and proinflammatory cytokines such as interleukin 1 and interleukin 8. These are all overexpressed following irradiation and lead to uncontrolled matrix accumulation and fibrosis.
In the proliferative phase overexpression of additional cytokines (e.g., epidermal growth factor [EGF], fibroblast growth factor [FGF], and platelet-derived growth factor [PDGF]) leads to dysregulated granulation tissue formation, re-epithelialization, and neovascularization.
Matrix metalloproteinases (MMPs) are responsible for the remodeling phase and regulate extracellular matrix synthesis. Irradiation can lead to dysregulation of MMPs leading to disorganized collagen deposition by fibroblasts.
There has been much interest in the postoperative complication rates of reconstructive surgery after radiotherapy and the optimal timing of surgery and radiotherapy. Much of the published literature is in reconstructive head and neck surgery, breast surgery, and resection following preoperative radiotherapy for rectal cancer.
It is understood that previously irradiated patients have a higher risk of postoperative complications. In cases of microvascular free flap transfer TGF-β1 has been shown to be over-expressed in free flaps for as long as 6 months after radiotherapy. Extracellular matrix remodelling has also been detected in the free flap for up to 5 months after radiotherapy completion. The alteration in graft bed extracellular matrix organization after radiotherapy and prior to surgery has been shown to impact significantly on free flap survival in the pre-irradiated field.
In head and neck reconstructive surgery, a large systematic review and meta-analysis combined data from 24 studies to compare outcomes for 2842 flaps performed in irradiated fields with 3491 flaps in nonirradiated fields. It was able to demonstrate that preoperative radiotherapy is associated with an increased risk of flap complications (RR 1.84, P <0.001), flap failure (RR 1.48, P <0.004), fistula formation (RR 2.05, P <0.001), and need for reoperation (RR 2.06, P <0.001).
Where radiotherapy is required as multimodality therapy the timing of radiotherapy has been the subject of much debate with regards to the risk of delayed wound healing and reducing the success of microvascular free flaps when radiotherapy is used preoperatively.
This has been retrospectively investigated for sarcoma patients, where 119 patients with sarcomas of the head and neck, upper and lower extremities were reviewed, with approximately two-thirds receiving preoperative radiotherapy and one-third receiving postoperative radiotherapy. Free flap loss rates were significantly lower in the preoperative radiotherapy group (0% vs. 8.7%). Late recipient site complications also occurred less often in patients receiving preoperative radiotherapy (6.8%) compared to postoperative radiotherapy (26.1%). A phase III Canadian study randomized 190 patients with soft tissue sarcoma of the extremities to either 50 Gy preoperatively or 66 Gy postoperatively. The study was closed early due to higher rates of wound complications in the preoperative group. Subsequent follow-up, however, demonstrated higher rates of grade 2–4 late toxicity in the postoperative radiotherapy group, with equivalent local control. On balance it is now generally felt preferable to accept a higher rate of acute, reversible toxicity with preoperative radiotherapy in a bid to reduce the incidence of irreversible late effects observed following postoperative radiation.
A review of 140 patients at the M.D. Anderson Cancer Center who underwent mandibular resection and reconstruction with a free fibular flap compared preoperative radiotherapy and immediate reconstruction, preoperative radiotherapy, and delayed reconstruction, postoperative radiotherapy, and no radiotherapy. Complications included orocutaneous fistula, osteoradionecrosis, and partial or complete flap loss. Forty-two percent of all patients studied had at least one complication, but the incidence of complications was relatively equal between the groups receiving pre- and postoperative radiotherapy.
Our practice in cases of preoperative radiotherapy for head and neck malignancies is to recommend surgery takes place within 6 weeks of completion of radiotherapy. Published data supports this approach, showing an increase in flap loss, infections, and delayed wound healing when more than 6 weeks elapses from the last radiotherapy session and surgery.
In breast cancer where there is a need for postmastectomy radiotherapy (PMRT), the optimal type and timing of reconstructive surgery also remains a controversial topic. Reconstructive options include a prosthetic device (e.g., silicone or saline implants), autologous tissue (e.g., transverse rectus abdominis musculocutaneous flap) or a combination of both. Reconstruction can be immediate at the time of mastectomy, “immediate-delayed” as a two-stage procedure usually completed within a number of weeks, or delayed.
In those requiring PMRT, prosthetic implant-based reconstruction is generally discouraged due to risks of reconstruction failure. A large systematic review demonstrated implant-based reconstruction failure rates of approximately 20% irrespective of whether undertaken pre- or postradiotherapy. Autologous flaps are also susceptible to radiation-related tissue damage, including fat necrosis, fibrosis, contracture, and loss of volume over time.
Current National Comprehensive Cancer Network (NCCN) guidelines recommend that women undergoing implant-based reconstruction undergo initial reconstruction with a two-stage approach using a temporary tissue expander and that women planned for an autologous reconstruction are delayed until radiotherapy has been completed. Practice trends are evolving, however, and the optimal strategy will always need to consider patient suitability for reconstructive options and personal preference.
In rectal cancer preoperative chemoradiotherapy is the current standard of care for T3–4 tumors and in selected patients with clinically node-positive disease, distal tumors or threatened mesorectal fascia involvement. The NCCN recommend surgical resection between 5 and 12 weeks post completion of radiotherapy, though optimal timing is yet to be determined. Surgical resection beyond 6 weeks may improve the proportion of patients achieving a pathological complete response (pCR) at resection, however delays risk tumor cell repopulation and may make surgical resection more difficult with an increase in postoperative complications. A large retrospective meta-analysis that included 3584 patients suggested that delaying surgery beyond 8 weeks increased the pCR rate by 6% but there was no evidence that long-term outcomes including overall survival were improved. Complication rates were similar.
Supportive Management
Many of the early effects of radiotherapy are evident during a treatment course. Patients are given written advice on skin care management, which includes the use of a daily emollient applied after each fraction of radiotherapy. Avoidance of direct sunlight on the treated area is imperative, as is avoiding the use of potentially irritating chemical-containing compounds on the skin (e.g., body lotions, perfumes, talcum powders, etc.). If moist desquamation develops emollients are not helpful and paraffin gauze dressings may offer some comfort. Early detection and treatment of superadded skin infections with oral antimicrobials is recommended.
Oral mucositis often requires analgesia. Optimal pain control is essential to ensure patients are able to take adequate oral nutrition. Analgesia is given stepwise according to need using the World Health Organization (WHO) analgesic ladder. Analgesic mouthwashes can also be used. Early dietetic and speech and language assessment of these patients is important, and if necessary parenteral feeding may be used if there is insufficient oral intake.
Xerostomia can be experienced early in the treatment course and is a well-documented late effect of radiotherapy after treatment of the salivary glands in head and neck malignancies. There are limited satisfactory treatment options to ameliorate this symptom. Artificial saliva sprays and gels may offer some relief. Due to the potential significant consequences of xerostomia on quality of life, which include increased rates of dental caries and oral candidiasis, and difficulty chewing and speaking, parotid-sparing radiation techniques are now used such as IMRT. Amifostine (a radioprotective agent) has also been shown to partially preserve parotid function after radiotherapy though it is not used in routine practice.
The management of late radionecrosis involves optimal wound care: adequate debriding and cleansing, accurately assessing the wound, choosing the appropriate dressing based on the wound assessment, and encouraging granulation tissue formation and re-epithelialization. Alginates, foams, gauzes, hydrogels, hydrocolloids, and transparent films are the major moisture-retentive dressings available.
HBOT can be used to treat osteoradionecrosis and radionecrotic wounds. Standard wound care should also be followed.
The aim of HBOT is to reverse the radiation-induced hypocellular, hypovascular and hypoxic tissue environment. HBO consists of exposing a patient to intermittent short-term 100% oxygen inhalation at a pressure greater than 1 atmosphere. HBO increases the oxygen concentration gradient so that the area around the necrosed hypoxic tissue has a high concentration of oxygen. This stimulates angiogenesis and reverses the hypoperfusion and hypoxia, giving tissues the propensity to heal. Initial reports of the benefit of HBO therapy were published as early as the 1970s. More recently a Cochrane Database Systematic Review concluded that for patients with late radiation tissue injury affecting the head, neck, anus, and rectum HBOT is associated with improved outcome.
Early studies of pentoxifylline in combination with tocopherol have been shown to be efficacious in reversing radiation-induced fibrosis. Pentoxifylline may also have a role in the treatment of ORN. This oral drug has an anti-TNF effect and vasodilator action, which improves microcirculation and enhances oxygen delivery to tissues. Encouraging results have been shown in a small phase II study combining pentoxifylline with tocopherol (vitamin E) and clodronate for the treatment of ORN of the mandible, with all patients experiencing complete recovery within a median of 9 months.
As the molecular basis of late radiation-induced tissue damage is now better understood, in particularly the specific cytokines central to the process, experimental molecular targets for therapeutic intervention are evolving. Fat grafting has been used in reconstructive surgery for years. There is now growing interest in the regenerative potential of adipose-derived stem cells (ADSCs) with their capacity for multilineage differentiation and ability to activate local stem cell niches. Alongside injection of multipotent cells, topical administration of active substances to reduce the side effects of radiotherapy and the use of growth factors (e.g., TGF-β1, 2, and 3), basic FGF, PDGF, and VEGF are all areas for future development.
Carcinogenesis
There is much evidence to support the carcinogenic property of ionizing radiation, namely studies from atomic bomb survivors in Japan, the Chernobyl nuclear accident, and people treated with high doses of radiation for cancer or other, benign conditions. In atomic bomb survivors the malignancies seen were leukemias and carcinomas of cells that line the body. There was no increase in the incidence of sarcomas (soft tissue tumors) in these patients. The risk of developing a radiation-induced malignancy varied significantly with age, with young children found to be 15 times as sensitive as middle-aged adults.
A contrast is seen with second malignancies induced by radiotherapy. These cancers are of cells lining the body but are often seen in tissues or organs not directly targeted and that therefore have received lower doses of radiation. Sarcomas are noted to arise in high-dose areas of radiation that lie within or immediately adjacent to the radiation fields ( Fig. 12.2 ).
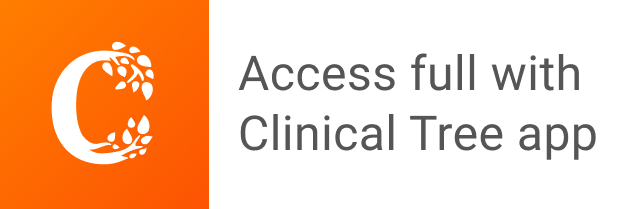