Abstract
Photodynamic therapy (PDT) is a unique, practical and effective therapeutic option for the treatment of actinic keratosis (AKs), superficial keratinocyte carcinomas, and a wide range of other conditions. Its photochemical reactions require photosensitizing molecules, photoactivating wavelengths, and tissue oxygen. In dermatology, PDT photosensitizers are mostly delivered topically as prodrugs (e.g. aminolevulinic acid and its congeners) that are metabolized in situ , i.e. within the skin, to generate photoactive protoporphyrin IX. Non-coherent artificial light (blue or red) can be used to illuminate the skin and energize the photosensitizer, which in turn transfers its energy to molecular oxygen, yielding singlet oxygen locally. Common adverse events of PDT include pain, photosensitivity, and inflammation. A recent innovation in PDT has been the use of daylight activation for the treatment of actinic keratoses. Pain during illumination is the major drawback with PDT, and daylight drug activation can significantly decrease its intensity.
Keywords
photodynamic therapy, PDT, protoporphyrin IX, aminolevulinic acid, ALA, methyl aminolevulinate, mALA, actinic keratosis, keratinocyte carcinoma, acne, photoaging
- ▪
Over the past two decades, photodynamic therapy (PDT) has evolved into a practical and effective therapeutic option, especially for actinic keratoses (AKs) and superficial non-melanoma skin cancers, with an expanding range of applications including acne, photoaging, cutaneous infections, and vascular anomalies
- ▪
The photodynamic effect is due to a photochemical reaction that generates singlet oxygen and it requires three components: photosensitizing molecules, photoactivating wavelengths of light, and tissue oxygen
- ▪
Singlet oxygen is highly reactive and initiates a series of cellular and tissue reactions that culminate in necrosis, apoptosis, and/or antimicrobial effects; host immunologic responses to singlet oxygen-altered tissue may also contribute to the overall clinical effect
- ▪
For cutaneous indications, the current standard technique is topical application of pro-photosensitizers (e.g. aminolevulinic acid) that are metabolized in situ , i.e. within the skin, to photoactive protoporphyrin IX
- ▪
Non-coherent artificial light (blue or red), daylight, or other light sources can be used to illuminate the skin and energize the photosensitizer
Introduction
Photodynamic therapy (PDT) involves the sequential administration of a photosensitizing agent followed by its activation utilizing light, which in turn generates singlet oxygen within biologic tissues ( Fig. 135.1 ). Singlet oxygen is a highly reactive form of oxygen that can induce necrosis and/or apoptosis and modulates a variety of other biologic processes. During the latter part of the twentieth century, PDT was synonymous with the use of systemic photosensitizers in combination with red laser light in order to treat cancers. Within dermatology, PDT evolved to applying a topical photosensitizer precursor to the skin and then illuminating the treatment site with artificial blue or red light. More recently, daylight activation of topical photosensitizers has been introduced, with an increase in treatment convenience and decrease in PDT-related pain.

History and Development
The photodynamic effect was originally studied in the early 1900s by a German medical student, Oscar Raab, who noted that cultures of Paramecium would die only if exposed to both an organic dye (acridine) and visible light. His professor, Dr Von Tappeiner, discovered the oxygen-dependence of this effect and coined the term “photodynamic action” to describe the phenomenon. In humans, hematoporphyrin was shown by Meyer Betz to induce profound and persistent generalized photosensitivity, including when he self-injected this agent. By capitalizing on its fluorescent properties when illuminated with a Wood’s lamp (365 nm peak emission), hematoporphyrin was shown to not only localize to neoplastic tissue, but to also induce tumor involution when exposed to visible light. Hematoporphyrin derivative was subsequently developed by Schwartz in an attempt to improve upon the tumor-localizing properties of hematoporphyrin, and then it was utilized by his colleague Lipson in animal models .
The mid 1970s marked the beginning of the modern era of clinical PDT when Dougherty and colleagues combined systemically administered hematoporphyrin derivative with visible light to treat cutaneous and subcutaneous malignancies in humans; they also confirmed the role of singlet oxygen . With the advent of lasers and fiberoptic-equipped endoscopes, the use of PDT was extended to carcinomas of the lung, bladder, and esophagus. Although the skin is obviously relatively easy to illuminate with light, hematoporphyrin derivative and its more purified, commercially available form (porfimer sodium [Photofrin ® ]) require intravenous administration and induce marked generalized cutaneous photosensitivity that lasts 6–8 weeks, necessitating strict avoidance of outdoor light. Second-generation porphyrin photosensitizers were then developed with pharmacokinetic profiles that favored more rapid elimination from the skin. This resulted in shorter periods of generalized photosensitivity, e.g. 1–3 days in the case of verteporfin . Although currently there is minimal use of systemic porphyrins to treat skin diseases, dermatologists should nonetheless be familiar with their medical use given their propensity to cause phototoxic cutaneous drug reactions ( Fig. 135.2 ).

Because they are relatively large molecules, the percutaneous absorption of porphyrins is rather poor. The breakthrough in developing PDT as a practical treatment option in dermatology can be attributed to the Canadian team of Kennedy and Pottier, who noted in the 1980s that a naturally occurring porphyrin precursor, 5-aminolevulinic acid (ALA), could photosensitize the skin by inducing local intracutaneous production of protoporphyrin IX (PpIX; see Fig. 135.1 ). Their successful treatment of non-melanoma skin cancers with topical ALA plus broadband red light abrogated the two major disadvantages associated with systemic photosensitizers (see above). The elegance of using topical ALA for PDT consists of how it takes advantage of the endogenous porphyrin biosynthetic pathway (which is present in all nucleated cells) in order to generate PpIX from ALA. The simplicity of this approach greatly facilitated clinical research, with the bulk of clinical studies and experience emanating, to date, from Europe. The use of topical ALA plus blue fluorescent light for the treatment of actinic keratoses (AKs) was approved by the US Food and Drug Administration (FDA) in 1999, just one decade after the first original publication by Kennedy and colleagues . In 2004, topical methyl aminolevulinate (mALA), an esterified congener of ALA, in combination with red light-emitting diode (LED) light, was approved in a number of countries for dermatologic PDT.
PDT – Photochemistry, Photobiology, and Mechanism of Action
PDT is somewhat analogous to PUVA (psoralen plus UVA light) therapy to the extent that both of these treatment modalities involve the administration of photosensitizers followed by exposure to specific wavelengths of light. Mechanistically, both involve exogenous photosensitizers that transfer photon energy from light to drive specific photochemical reactions within the skin. Nevertheless, there are important differences between PDT and PUVA ( Table 135.1 ), the most fundamental of which is the fact that, by definition, PDT generates singlet oxygen, which in turn is biologically active.
PHOTODYNAMIC THERAPY VERSUS PUVA THERAPY | ||
---|---|---|
Features | PDT | PUVA |
Routes of administration of photosensitizer | Topical Systemic (IV) | Topical Systemic (PO) |
Photosensitizer | ALA, mALA (prodrug) | 8-methoxypsoralen |
Active form of photosensitizer | PpIX | 8-methoxypsoralen |
Photosensitizer activation | Visible light | Ultraviolet A |
Photochemical reaction | Type II: convert O 2 to singlet O 2 | Type I: covalent adducts between psoralen and DNA; non-oxygen-dependent |
Relationship with skin cancer | Treats keratinocyte carcinomas | Carcinogenic |
Effects of long-term use | Generally safe, can be used to treat photoaging | Skin cancer, photoaging |
PDT Photochemistry
The transfer of energy from light to tissue can be illustrated diagrammatically by considering the resting and excited states of the molecules involved in photochemical reactions ( Fig. 135.3 ). The photosensitizer is delivered to or generated within the target tissue in its ground, lower-energy resting state. Its efficiency as a chromophore for absorbing light energy is governed by its absorption spectrum ( Fig. 135.4 ). If light of an appropriate wavelength is used to irradiate the photosensitizer within tissue, photon absorption occurs and the photosensitizer is excited to a higher-energy “singlet” state. The excited singlet is short-lived, lasting nanoseconds, and then undergoes intersystem crossing to a more stable “triplet” state, which has a lifetime on the order of microseconds. The “triplet” photosensitizer species can transfer its stored energy to molecular oxygen, yielding singlet oxygen ( 1 O 2 ), and, as a result, returns to its resting ground state. Photochemical reactions that are mediated by oxygen are referred to as type II photochemical or photosensitized reactions. The singlet oxygen thus generated reacts with a variety of cellular components, including lipids, proteins, and nucleic acids.


Singlet oxygen is by no means the only active intermediate generated by the excited triplet photosensitizer. A variety of other reactive oxygen species, including peroxides (H 2 O 2 ), superoxide anion (O 2 − ) and hydroxyl radicals (.OH), are generated from molecular oxygen and undoubtedly contribute to the overall biologic effect. Singlet oxygen and these other reactive oxygen species can also interact chemically with the photosensitizer itself, leading to its inactivation by a process known as photobleaching . In this way, the PDT process can in part shut itself off automatically.
Light within the visible spectrum is typically used for PDT. This is because ultraviolet light has limited tissue penetration, and infrared radiation (>850 nm) does not possess sufficient photon energy to trigger the transformation of molecular oxygen into singlet oxygen via an excited photosensitizer intermediary.
When PDT photosensitizers are excited to their singlet states following light absorption, they can alternatively return to their ground state via the emission of photons. This “re-emission” of light by the photosensitizer is termed fluorescence and the latter property permits, with Wood’s lamp illumination, direct visualization of photosensitizer accumulation within tissue as well as its subsequent depletion due to photobleaching following light exposure.
Photobiologic Mechanisms of Action
Although the exact and most relevant molecular sequence of reactions initiated by singlet oxygen has not been determined, oxidative damage (including to cellular membranes) is known to trigger cellular injury and apoptosis. For a given PDT clinical effect or indication, the specific mechanism of action depends on the target tissue, the photosensitizer and its mode of delivery, subcellular photosensitizer localization, and the overall drug–light dose delivered. For example, when topical ALA is applied to an epidermal neoplasm such as a superficial basal cell carcinoma (BCC), PpIX accumulates within the tumor cells themselves, with direct cytotoxicity upon light activation (see Fig. 135.1 ). In contrast, systemic photosensitizers are known to preferentially localize to the vascular bed of tumors. Light activation then leads to vascular collapse and ischemic tumor necrosis .
At a high drug–light dose combination, greater levels of singlet oxygen are generated during PDT, leading to necrotic tissue damage. With lower levels of drug and/or light, damage is more limited, with apoptotic changes in a fraction of the cells. Because PDT is oxygen-dependent, target tissues that are hypoxic may be relatively resistant to the desired therapeutic effect.
Tissues exposed to PDT can become inflamed as a result of normal host responses to injury as well as activation of specific immunologic reactions. However, the type of immunologic reaction varies depending upon the target and mode of PDT delivery. In animal models of contact hypersensitivity, PDT suppresses local and systemic immune responses in addition to reducing epidermal Langerhans cells . There is also evidence that the photosensitizers used in PDT can target activated T cells , providing a rationale for using PDT to treat disorders such as psoriasis or mycosis fungoides. Microbes, including viruses, bacteria, fungi and parasites, may be susceptible to singlet oxygen, and there is a number of clinical studies attesting to the potential utility of PDT in the treatment of skin infections.
PDT Selectivity
PDT was initially conceived and developed as a method for achieving selective tumor destruction. Several factors lead to this selectivity ( Table 135.2 ). In neoplasms, where cellular metabolism is higher than normal, local factors such as enhanced perfusion, vascular and lymphatic hyperpermeability, pH, and lipoprotein receptors on target cells may lead to preferential accumulation and retention of photosensitizers within the tumor. With topical PDT, the specific site of drug application is set by the physician, thereby delimiting the zone of photosensitization and treatment. There are also inherent differences in the permeability barrier of skin lesions such as BCCs and AKs that facilitate the selective uptake of topical ALA-like prodrugs into diseased skin.
THERAPEUTIC SELECTIVITY DURING PHOTODYNAMIC THERAPY | |
---|---|
Photosensitizer effects | Light effects |
|
|
Selectivity is also achieved by determining the manner in which PDT light is delivered (see Table 135.2 ). This involves limiting the area of light exposure to just the specific skin target. In other words, the lesion or lesions (plus a limited margin of adjacent normal-appearing skin) is typically located in the center of the exposure field. Most clinical photosensitizers exhibit multiple absorption maxima across the visible spectrum (see Fig. 135.4 ). Because light propagation within tissue is wavelength-dependent, the use of shorter wavelengths, i.e. blue light, will confine the PDT effect to primarily the epidermis, while red light will exert a deeper effect. For deeper targets within the skin, fiberoptic probes can be implanted interstitially in the dermis or subcutaneous tissue to deliver light.
Photobleaching is another mechanism by which PDT can exert therapeutic selectivity. A certain threshold “PDT dose” is necessary for effects such as tissue necrosis via singlet oxygen. Within the target site, where the photosensitizer concentration is presumably above this required threshold, singlet oxygen generation will prevail. In contrast, within normal tissue where the photosensitizer level is below this threshold, light exposure will predominantly cause photobleaching rather than generating sufficient singlet oxygen to induce tissue damage.
Photosensitizer selectivity can be readily visualized clinically because PDT photosensitizers usually have high fluorescence quantum yields that are apparent with Wood’s light examination ( Fig. 135.5 ). However, fluorescence intensity does not necessarily correlate with the ensuing PDT reaction or ultimate clinical outcome. Immediately, at the conclusion of the light exposure stage, the phenomenon of photobleaching can be confirmed visually by noting the absence or marked reduction of fluorescence.

Advantages and Limitations of PDT
Advantages of PDT include its relative conceptual simplicity as well as the accessibility of the skin to direct application of photosensitizing drugs and activating light. PDT photosensitizers typically have minimal dark toxicity (i.e. negligible toxicity in the absence of light) and are therefore relatively safe. PDT can also be used in patients with a variety of comorbidities, including those who are anticoagulated; general anesthesia is rarely required or even used for cutaneous PDT. PDT treatments can also be repeated and there are minimal long-term side effects. Additionally, it has the advantage of being a physician-supervised therapy, which limits the effects of poor compliance as can be seen with at-home topical treatments.
Nonetheless, PDT is far from being a perfect therapeutic modality as it has inherent limitations. The generation of singlet oxygen within the target site is critically dependent on the ability to deliver both photosensitizer and light to this site in the presence of oxygen. Topical photosensitization is limited by drug penetration into the epidermis and dermis, while the depth of penetration of visible light limits its delivery when administered by surface illumination. Although infrared wavelengths are longer and more penetrating, they do not have sufficient photon energy to generate singlet oxygen. Thus, PDT is useful primarily for cutaneous tumors and diseases that are superficial.
The fact that there are multiple treatment parameters can also be a disadvantage in that the multiplicity of combinations and permutations makes it complicated to determine the conditions necessary for optimal clinical outcomes. Moreover, the literature is replete with an array of different reported parameters, making it difficult to standardize treatment regimens and evaluate clinical evidence of efficacy, even for the same indication. The commercially recommended treatment parameters are based on strict clinical trial protocols and do not afford much flexibility in terms of dosing. From the patient perspective, intraoperative local pain at the site of artificial light exposure can be intense and even intolerable, and for many patients this represents a significant disincentive for repeat treatments. Commercially available PDT systems (i.e. photosensitizers and artificial light sources) incur significant cost, thereby posing a practical barrier to accessing a treatment that is fundamentally based on a naturally occurring compound combined with visible light. Natural daylight activation of photosensitizers can partially circumvent the costs and apparent complexity of administering PDT.
Components of PDT
A wide range of photosensitizers have been developed and evaluated along with a multiplicity of different light sources and delivery systems ( Table 135.3 ). Of these, two commercial systems have been approved by the FDA: ALA plus blue light and mALA plus red light, with additional methods being available in other countries.
COMPARISON OF ARTIFICIAL LIGHT-ACTIVATED PDT, DAYLIGHT-ACTIVATED PDT, AND ALTERNATIVE TECHNIQUES FOR PDT | |||
---|---|---|---|
Feature | Artificial light-activated PDT | Daylight-activated PDT (D-PDT) | Technique variations of PDT (off-label) |
Lesion preparation |
|
|
|
Drug delivery |
|
|
|
Photosensitizer |
|
| |
Incubation time before light exposure |
|
| |
Light activation |
| Light sources:
Light delivery:
| |
Pain |
|
|
|
** Approved in multiple European countries.
*** Approved in the UK, multiple European countries, and Australia.
Photosensitizers
Although topical and systemic photosensitizers based on the full porphyrin backbone structure have been developed and evaluated, to date none have become practical, on a clinical or commercial level, for dermatologic use. For practical purposes, topical PDT relies primarily on the “ALA prodrug” approach.
ALA and mALA represent topical prodrugs that are converted within the skin into PpIX. A familiarity with porphyrinogenesis in mammalians aids in understanding the pharmacology of ALA and its congeners. ALA (5-aminolevulinic acid, δ-aminolevulinic acid) is synthesized in all nucleated cells, and it is an intermediate compound in the heme biosynthetic pathway (see Fig. 49.1 ); ALA synthase catalyzes the formation of ALA from succinyl-CoA and glycine. The latter reaction represents the rate-limiting step in this pathway and is inhibited by heme.
When pharmacologic quantities of ALA are supplied to cells as in PDT, this regulatory step is bypassed, leading to an intracellular accumulation of PpIX, which is photodynamically active. Of note, PpIX is synthesized within mitochondria (see Fig. 135.1 ) and then diffuses to cellular membrane systems such as the endoplasmic reticulum and the plasma membrane.
Unlike fully formed porphyrin molecules that are relatively large, ALA is small and readily crosses the cutaneous permeability barrier via active transport mechanisms. Within the skin, the absorbed ALA induces porphyrinogenesis, thereby inducing “porphyria” at the cellular level. In some PDT applications, the skin is first prepared by gentle curettage of excess surface scale and crust, and this may also promote topical drug absorption. Within the skin, PpIX accumulates in the epidermis and sebaceous glands due to its lipophilicity.
The carboxyl moiety of ALA can be esterified by a variety of alkyl groups, the simplest of which results in mALA. Substituted ALA congeners are absorbed into the skin by passive and active mechanisms different from those of ALA. Upon their uptake into cells, these substituents are hydrolyzed to yield free ALA (see Fig. 135.1 ). Both ALA and mALA are considered prodrugs or pro-photosensitizers since the skin itself actually generates the active photosensitizer PpIX following application of these exogenous compounds.
ALA has been compounded in a variety of different vehicles for topical application and, in North America, it is currently commercially available in a 20% preparation. The latter is a topical hydroalcoholic solution that is freshly prepared immediately prior to application. Its approved indication is for treating AKs, although multiple off-label uses have been explored and described ( Table 135.4 ). Time is required to allow ALA to be absorbed and converted to PpIX. When utilizing artificial light with ALA, many clinicians typically use a shorter incubation period of 1–4 hours, instead of the recommended 14–18 hours employed in the original controlled clinical trials.
PHOTODYNAMIC THERAPY – DERMATOLOGIC APPLICATIONS |
FDA-approved indications |
Actinic keratoses of the face and scalp (ALA solution or gel with blue or red light, respectively; mALA with red light) |
Off-label * applications with controlled studies demonstrating efficacy |
Actinic keratoses (including D-PDT and PDT of sites beyond the face and scalp) Superficial basal cell carcinoma Squamous cell carcinoma in situ (Bowen disease) Prevention of keratinocyte carcinomas Acne Photoaging Verrucae vulgares Condylomata acuminata Leishmaniasis |
Off-label applications for which PDT is not considered first-line therapy |
Actinic cheilitis Nodular basal cell carcinoma Lentigo maligna (melanoma in situ arising in photodamaged skin) Extramammary Paget disease Cutaneous metastases Capillary malformations (port-wine stains) Chronic ulcers Superficial fungal infections Hypertrophic scars/keloids |
Diseases for which PDT is not generally recommended |
High-risk basal cell carcinoma (e.g. micronodular, morpheaform) Invasive squamous cell carcinoma Invasive cutaneous melanoma |
Mechanistically, mALA is similar in principle to ALA; it is available in a 16.8% cream formulation that must be stored in a refrigerator. The recommendation is to apply mALA for 3–4 hours under occlusion prior to exposure to red light from an LED. It has been used for multiple different skin diseases, but depending upon the country, the approved indications are treatment of AKs and/or superficial keratinocyte carcinomas (see Table 135.4 ). Currently, mALA is no longer marketed in the US pending additional FDA-mandated post-marketing studies.
Newer ALA formulations have recently been developed, including a nanoemulsion gel and an ALA-impregnated adhesive topical patch, with the latter not requiring pretreatment with curettage .
Light Sources
Choosing a light source for PDT depends fundamentally on three considerations. First, the spectral output of the light should include a waveband that corresponds to one or more of the peaks in the absorption spectrum of the photosensitizer (see Fig. 135.4 ). Next, the desired depth of effect determines which photosensitizer absorption peak should be chosen. For PpIX, red light activation will provide a deeper clinical effect as compared to blue light. Finally, the surface area to be treated must be taken into account. For example, while lasers can provide precise irradiation, they may be less practical when treating broader, extensive surfaces.
Artificial light sources
Light emitting diodes (LEDs) and blue fluorescent lamps have been the most commonly employed artificial light sources for PDT (see Table 135.3 ). In general, these commercial devices are relatively simple to use and can be powered by standard electrical outlets.
Lasers (e.g. copper vapor, KTP and pulsed dye) may be as effective as non-coherent artificial lights for PDT ; however they are significantly more expensive, more complex to operate, and less studied. Intense pulsed light (IPL) has also be used as an artificial light source for PDT . Adhesive, light-emitting patches have been designed to be worn by patients and because they operate at low irradiances, there may be less pain associated with these devices .
Natural daylight
Recent studies have shown that natural daylight may be used for PDT drug activation since it contains all of the appropriate wavelengths. The main apprehension with daylight activation is the inability to accurately control the light dose delivered to the skin, with the possibility of phototoxicity from overexposure or lack of efficacy due to underexposure. However, randomized controlled trials have demonstrated both safety and efficacy with daylight-activated PDT (D-PDT; also referred to as DA-PDT). Specifically, non-inferiority for effective treatment of AKs and superiority in degree of associated pain were demonstrated in a split-face trial of daylight- versus red light-activated PDT .
Although not yet FDA-approved, D-PDT has the potential to transform the administration and practicality of PDT due to several advantages. There is no requirement for a specific topical drug incubation time since patients are typically exposed to outdoor light immediately after drug application. Patient self-exposure to ambient outdoor light eliminates the requirement for staff to operate a specialized device, and perhaps most importantly, the level of PDT-related pain is much reduced with D-PDT. Published measurements of daylight doses in various cities can help clinicians identify months in which there is sufficient outdoor light to effectively administer D-PDT in their locale . As a general rule, if the latitude is <45° (as in most of the US, southern Europe, South America, and Australia), PDT can be done year round depending on weather conditions . At higher latitudes in the northern hemisphere, it might be preferable to avoid daylight PDT from October to April. Of note, direct sunlight exposure to the skin is not necessary for daylight PDT, and chemical sunscreens, applied in order to provide protection from daylight UV exposure, do not interfere with the absorption of visible light by PpIX.
Light delivery
Regardless of the specific light source, the PDT light dose is measured and delivered in a manner analogous to conventional phototherapy. The irradiance of the lamp is specified in units of W/cm 2 , which represents the power density over a surface area unit. Irradiance depends on the light source itself, and it can vary based on the lamp’s age, overall operating conditions, and the distance from the lamp to the skin target. The absolute light dose used to activate the photosensitizer is also known as the fluence and is measured in J/cm 2 . The exposure time in seconds required to deliver a specified fluence can be determined from the formula:
exposure time = fluence ( J / c m 2 ) irradiance ( W / c m 2 )
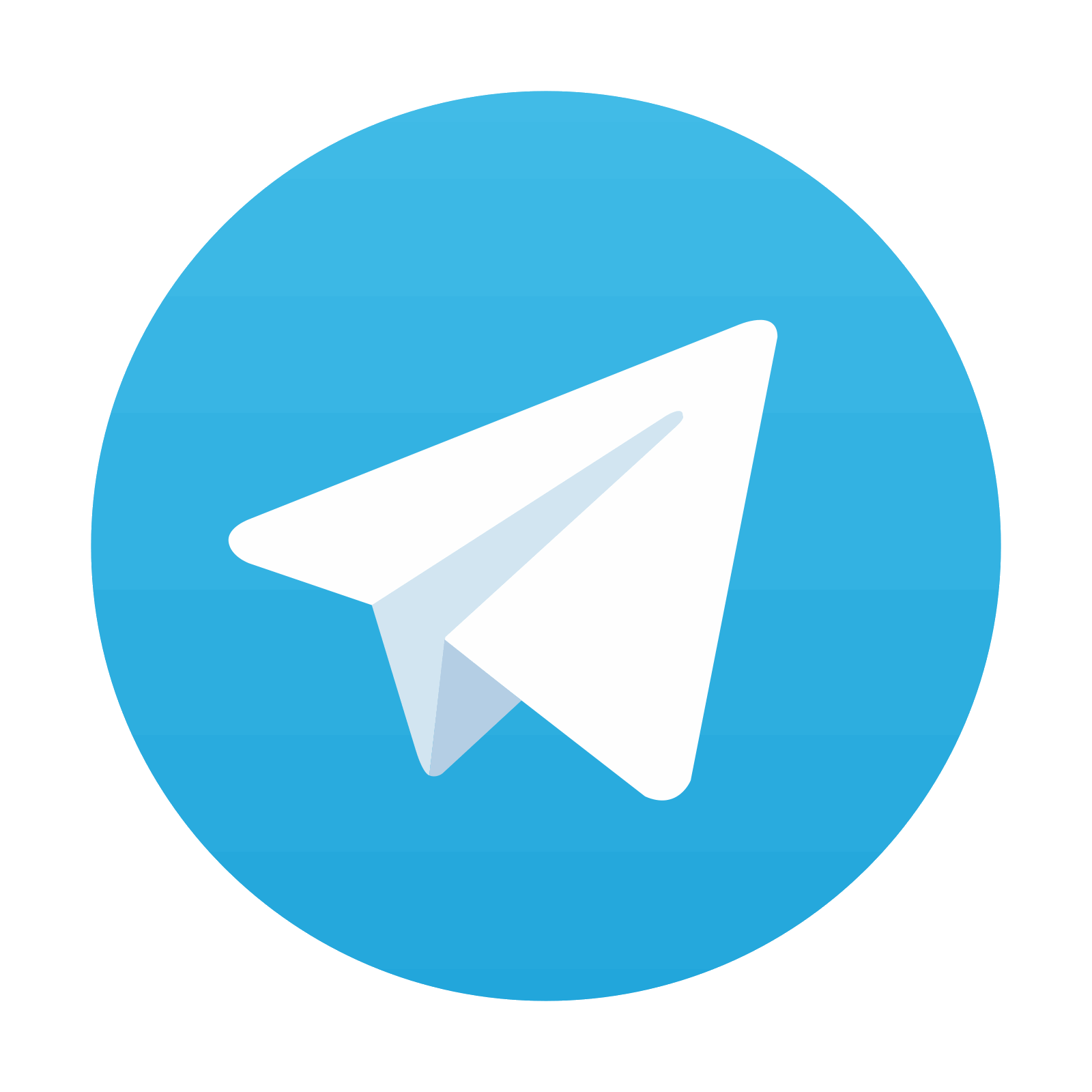
Stay updated, free articles. Join our Telegram channel
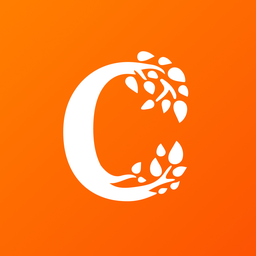
Full access? Get Clinical Tree
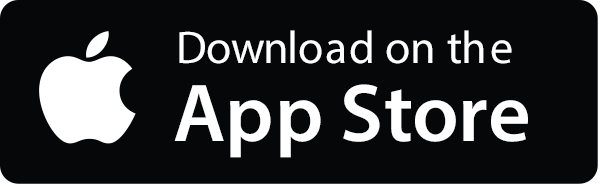
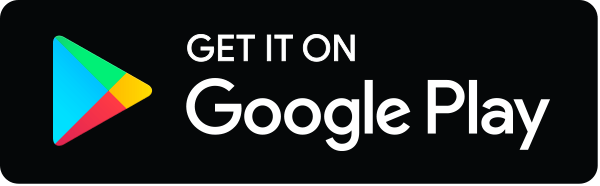