Abstract
Laser surgery is based upon precise tissue heating when optical energy is absorbed within the skin, and the heat is created exactly where light is absorbed by molecules called chromophores. The major chromophores for visible and near-infrared light are melanin and hemoglobins while water is the major far-infrared chromophore. Wavelength(s) of lasers or intense pulsed light (IPL) sources are often matched for absorption by chromophores in skin targets such as blood vessels, hair follicles, melanin-containing cells, tattoo ink, or tissue layers. Selective heating and damage of targets can occur when a pulse of selectively absorbed light is delivered faster than the targets can cool by heat conduction. The time for a tissue target to cool is its thermal relaxation time, equal in seconds to approximately the square of the target size in millimeters. Very short (nanosecond) laser pulses, produced by Q-switched lasers, can precisely damage small targets such as individual melanocytes. Millisecond lasers and IPL sources selectively affect multicellular targets such as blood vessels, hair follicles and tissue layers. In fractional laser treatments, an array of microscopic thermal injury zones is created. Additional energy technologies include radiofrequency electrical current and high-intensity, focused ultrasound.
Keywords
laser, chromophores, fractional laser, intense pulsed light, thermal relaxation time, selective photothermolysis, high-intensity focused ultrasound, thermal injury zone, microthermal zone of injury, Q-switched lasers
- ▪
Laser surgery works via precise tissue heating when optical energy is absorbed within the skin
- ▪
Heat is created exactly where light is absorbed by molecules called chromophores
- ▪
The major chromophores for visible and near-infrared light are melanin and hemoglobins; water is the major far-infrared chromophore
- ▪
Red and near-infrared wavelengths are deeply penetrating
- ▪
Wavelength(s) of lasers or intense pulsed light (IPL) sources are often matched for absorption by chromophores in skin targets such as blood vessels, hair follicles, melanin-containing cells, tattoo ink, or tissue layers
- ▪
Selective heating and damage of targets can occur when a pulse of selectively absorbed light is delivered faster than the targets can cool by heat conduction
- ▪
The time for a tissue target to cool is its thermal relaxation time, equal in seconds to approximately the square of the target size in millimeters
- ▪
Very short (nanosecond) laser pulses, produced by Q-switched lasers, and picosecond laser pulses can precisely damage small targets such as individual melanocytes and tattoo ink particles
- ▪
Millisecond lasers and IPL sources selectively affect multicellular targets such as blood vessels, hair follicles, and tissue layers
- ▪
Fractional laser treatments create an array of microscopic thermal injury zones
- ▪
Radiofrequency electrical current, traditionally used for skin surgery, has been re-configured for esthetic applications
- ▪
High-intensity, focused ultrasound can heat a precise focus within or below the skin
- ▪
By crystallizing lipids, controlled cooling (cryolipolysis) can reduce localized subcutaneous fat
- ▪
Laser microscopy of intact living skin is an emerging diagnostic tool
Introduction
The last century took us from Einstein’s insight into the quantum nature of light, to the invention of the ruby laser in 1960, to the wide spectrum of lasers available today. In particular, the principle of selective photothermolysis guided the development of pulsed lasers that target specific skin structures such as blood vessels, melanosomes, tattoo ink, and hair follicles. Subsequent advances included flashlamps, also referred to as intense pulsed light (IPL) sources, and fractional photothermolysis in which thousands of microscopic zones of thermal injury are created that stimulate skin remodeling. Additional energy-based technologies include focused ultrasound, radiofrequency electric current, and controlled skin cooling. The field of laser and other energy-based technologies is a dynamic one and serves as steady source of therapeutic innovation.
Lasers
LASER is an acronym for l ight a mplification by s timulated e mission of r adiation. It is a physical process that can be explained by Einstein’s principles of electromagnetic radiation . The quantum unit of electromagnetic energy is a photon, and stimulated emission occurs when one photon stimulates an excited atom to emit a second, identical photon. The idea of lasers was envisioned by Charles Townes , and in 1960 a laser utilizing a flashlamp-excited ruby crystal was first introduced . Soon thereafter, Dr Leon Goldman initiated dermatologic applications of laser technology.
All lasers have four essential components ( Fig. 136.1 ): (1) a gas, liquid or solid medium that can be excited to generate light ( Table 136.1 ); (2) a source of pump energy for exciting the medium; (3) mirrors at the ends of the lasers which form an optical cavity that favors the amplification process; and (4) a delivery system. Light is amplified as it travels back and forth between the laser’s mirrors thereby generating a very high intensity laser beam.

TYPES OF LASER MEDIA | ||
---|---|---|
Gas | Liquid | Solid |
| Rhodamine dye dissolved in organic solvent † |
|
Laser light has several properties that are different from other light sources. Monochromicity refers to emission of a well-defined wavelength, rather than a band of wavelengths. Coherence describes light waves that travel in phase, both in time and space, similar to a column of soldiers marching in step. Collimation refers to a very parallel beam of light. Coherence and collimation allow lasers to be focused to very small spot sizes (see Fig. 136.1 ).
Lasers may emit a continuous beam or pulses of light, with nearly all dermatologic lasers currently in use emitting pulsed light. Q-switched lasers produce short pulses at very high peak power. “Q” refers to a quality factor for light traveling across the laser cavity, which can be changed suddenly, i.e. “switched”, to produce a short, intense burst of light. Dermatologic Q-switched lasers are designed to produce pulses of ~1–100 nanoseconds, with fluence (energy density) typically in the 2–10 J/cm 2 range. These short, high-power pulses are particularly useful for selective removal of tattoos and pigmented lesions while limiting thermal damage to surrounding tissues . The repetition rate for pulsed lasers is expressed in hertz (Hz). Of note, some lasers emit a rapid train of low-energy pulses that behave surgically like continuous-wave lasers and are called quasicontinuous.
Skin Optics
At the skin surface, 4–7% of light is typically reflected because of the difference in the refractive index between air ( n = 0) and stratum corneum ( n = 1.45) . This is termed the Fresnel reflectance because it follows Fresnel’s equations relating reflectance to the angle of incidence, plane of polarization, and refractive index . The remaining 93–96% of incident light enters the skin, where it is scattered and/or absorbed ( Fig. 136.2 ). Scattering occurs when photons “bounce” off particles and fibers within the skin, leading to diffusion of the incoming beam of light and limiting its depth of penetration. The absorption coefficient is defined as the probability per unit path length that a photon at a particular wavelength will be absorbed, and it depends on the concentration of chromophores (absorbing molecules) present. After the energy of the photon is absorbed by the chromophore, then heat or photochemical reactions lead to tissue effects.

Three primary skin chromophores are water, hemoglobin and melanin ( Fig. 136.3 ). Chromophores exhibit characteristic bands of absorption at certain wavelengths. It is this fact that allows for the delineation of specific targets for different laser wavelengths. While melanin absorbs broadly across the visible and ultraviolet (UV) spectrum, the oxyhemoglobin and reduced hemoglobin in blood exhibit strong bands in the UV, blue, green and yellow regions. Water has strong absorption in the infrared (IR) region ( Fig. 136.4 ).


Optical properties of the epidermis and dermis are different. In pigmented epidermis, melanin absorption is usually the dominant process over the majority of the optical spectrum (200–1000 nm) (see Fig. 136.3 ). In the dermis, there is strong, wavelength-dependent scattering by collagen fibers, which attenuates penetration of light. This scattering varies inversely with wavelength. In general, between 280 and 1300 nm, the depth of penetration increases with wavelength ( Fig. 136.5 ). Above 1300 nm, penetration decreases due to the absorption of light by water. The most deeply penetrating wavelengths are 650–1200 nm, while the least penetrating wavelengths are within the UV and IR regions.

Thermal Interactions
Most laser interactions involve heat. As temperature is raised, essential macromolecules are denatured including proteins, DNA, RNA and cell membranes, leading to loss of function. Thermal coagulation yields cell necrosis and, if widespread, a burn. Laser skin surgery requires precise control over the placement and amount of heat-induced injury.
Most human cells can easily withstand temperatures up to 40°C. Cellular survival is dependent upon both exposure time and temperature. In most organisms, exposure to sub-lethal heat induces a cellular reaction termed the heat shock response. Normal protein synthesis is replaced by synthesis of proteins called heat shock proteins; the latter confer some resistance to thermal injury . Laser-induced thermal injury is well described by an Arrhenius model – the rate of denaturation is exponentially related to temperature. Thus, accumulation of denatured material rises exponentially with temperature and proportionally with time . Near a critical temperature (which is different for different tissues), rapid coagulation occurs; this is what accounts for the well-defined histologic boundaries of dermal coagulation in laser and other burn injuries. In the dermis, type I collagen plays a primary role in thermal coagulation, whereas elastin is extremely thermally stable and can survive boiling without apparent injury. Fibrillar type I dermal collagen has a sharp melting and shrinkage transition at 60–70°C. At or above this temperature range, scarring becomes more likely. Selective photothermolysis permits selected heating of targets within the dermis, such as blood vessels or hair follicles, with preservation of dermis between targets. However, even with selective photothermolysis, there is an absolute need to keep the bulk skin temperature well below ~60–70°C. Clinically, immediate skin responses can indicate localized thermal damage or excessive and non-selective unwanted damage .
When laser light, intense pulsed light (IPL), radiofrequency (RF), ultrasound, or other form of energy is absorbed by tissue, heat is produced locally which immediately begins to diffuse into the surrounding tissue. Heat diffusion via conduction is also called thermal relaxation. Thermal relaxation time (TRT) is defined, for a given tissue structure, as the time required for the heated tissue to cool halfway towards its initial temperature. The key to clean ablation of tissue is to ablate it quickly, before much heat is conducted into surrounding tissue .
Selective Photothermolysis
The concept of selective photothermolysis first arose to guide the design of a laser for treating pediatric port-wine stains. Selective, localized heating (with focal destruction of “target” structures) is achieved by a combination of selective light absorption and a pulse duration shorter than or approximately equal to the TRT of the targets . Choice of wavelength(s) must correspond to absorption by chromophores within the skin targets. The wavelength must also penetrate to the anatomic depth of the skin targets.
The TRT of a target is proportional to the square of its size ( Table 136.2 ). Thus, for a given material and shape, an object half the size will cool in one-quarter of the time. For most tissue structures, TRT (in seconds) is approximately equal to the square of the target dimension (in millimeters). Shape also affects TRT, and for a given thickness, planes cool faster than spheres. In general, the optimal pulse duration for selective photothermolysis is approximately equal to the TRT. Selective photothermolysis typically utilizes pulsed (rather than continuous) sources . Blood vessels encompass a wide range of TRTs, including capillaries (tens of microseconds), large venules of adult port-wine stains (up to tens of milliseconds), telangiectasias and leg veins (hundreds of milliseconds). Small pigmented targets (e.g. melanosomes within melanocytes in a nevus of Ota, tattoo ink particles within fibroblasts) are best treated with short (sub-microsecond) pulses, while larger pigmented targets (e.g. hair follicles) have longer TRTs and are best treated with longer (millisecond) pulses .
PULSE DURATIONS AND TARGETS OF SELECTIVE PHOTOTHERMOLYSIS | |||
---|---|---|---|
Chromophore | Diameter | TRT | Typical laser pulse duration |
Tattoo ink particle | 0.01–0.5 micron | 100 ps–250 ns | 300 ps–50 ns |
Melanosome | 0.5 micron | 250 ns | 10–100 ns |
PWS vessels | 30–100 microns | 1–10 ms | 0.4–20 ms |
Terminal hair follicle | 300 microns | 100 ms | 3–100 ms |
Leg vein | 1 mm | 1 s | 0.1 s |
Photomechanical Effects
Pulsed lasers can also cause mechanical effects . Sudden heating induces thermal expansion with consequent acoustic and/or shock waves that can rupture or increase permeability of cell membranes. Cavitation, the violent formation and collapse of steam bubbles, may also occur. Examples of mechanical injury are purpura due to vessel rupture from pulsed dye lasers emitting pulses less than ~10 milliseconds and tattoo ink particle fracture from lasers emitting picosecond pulses.
A number of parameters control laser-tissue effects, including wavelength, fluence, irradiance, spot size, and pulse duration ( Table 136.3 ). With smaller spot sizes, scattering leads to a greater loss of photons from the beam as it penetrates the skin, as compared to larger spot sizes. The greatest effective penetration depth into skin occurs with the combination of a penetrating wavelength (600–1300 nm; see Fig. 136.5 ) and a large spot size.
USEFUL LASER TERMS | ||
---|---|---|
Term | Definition | Unit |
Energy | Fundamental unit of work | Joules (J) |
Power | Rate at which energy is delivered | Watts (W) |
Fluence | Amount of energy delivered per unit area | J/cm 2 |
Irradiance | Power delivered per unit area | W/cm 2 |
Pulse duration (pulse width) | Laser exposure duration | Seconds (s) |
Spot size | Diameter of the laser beam on the skin surface | mm |
Chromophore | Medium that absorbs light | |
Thermal relaxation time | Time required for heated tissue to lose 50% of its heat through diffusion | Seconds (s) |
Cooling for Skin Protection
Epidermal melanin is an undesired chromophore when treating dermal targets. Epidermal damage can be minimized through the use of skin cooling, which is especially important with darkly pigmented skin. All cooling methods extract heat at the skin surface via a cooling agent (gas, liquid or solid). For dynamic cryogen spray cooling, a liquid fluorocarbon is sprayed onto the skin. Solid contact cooling typically consists of a cold sapphire window held against the skin, through which laser or IPL energy is delivered. Cold air or cold gels can also be applied for passive skin cooling.
The terms precooling, parallel cooling and postcooling refer to cooling before, during and after the laser exposure, respectively . For pulse durations shorter than ~5 milliseconds (e.g. from many pulsed dye and all Q-switched lasers), there is not enough time for parallel cooling to extract much heat, but precooling provides some protection. For short pulsed lasers, cryogen spray cooling represents the most aggressive and effective form of precooling. Parallel cooling is effective for pulses longer than ~5 milliseconds. Bulk skin cooling before and after laser treatments (e.g. with ice or cold air) is useful for minimizing pain, erythema, and edema .
Applications of Laser Principles
Currently available medical lasers are outlined in Table 136.4 and their applications are discussed in Chapter 137 .
LASERS IN MEDICINE | |||||||
---|---|---|---|---|---|---|---|
Laser | Wavelength (nm) | Mode | Average power (W) | Average energy per pulse (J) | Pulse duration (sec) | Pulse rep. rate (Hz) | Target chromophore |
Excimer (ArF) | 193 | Pulsed | 10 | 0.1 | 10 −8 | 100 | Proteins |
Excimer (XeCl) | 308 | Pulsed | 10 | 0.1 | 10 −7 | 100 | Proteins |
Argon | 488, 514 | CW | 1–10 | Hemoglobins, melanin | |||
Copper vapor | 511, 578 | Quasi-CW | 1–10 | 10 −3 | 10 −8 | 2 × 10 3 | Hemoglobins, melanin |
KTP | 532 | Quasi-CW | 1–10 | 10 −4 | 10 −8 | 104 | Hemoglobins, melanin |
Q-switched, frequency-doubled Nd:YAG | 532 | Pulsed (ns) | 1–3 | 0.2 −1 | 10 −8 | 5–10 | Melanin, red tattoos |
Pulsed dye | 585–600 | Pulsed | 10 | 5 | (0.45–40) × 10 −3 | 2 | Hemoglobins |
Argon dye | 630 | CW | 0.5–2 | Photosensitizing drugs | |||
Ruby | 694 | Pulsed | 30 | 30 | 10 −3 | 1 | Melanin |
Q-switched ruby | 694 | Pulsed (ns) | 2 | 2 | 3 × 10 −8 | 1 | Melanin, black and green tattoos |
Alexandrite | 755 | Pulsed | 30 | 30 | 10 −3 | 1 | Melanin, deoxyhemoglobin |
Q-switched alexandrite | 755 | Pulsed | 5 | 0.5–5 | 10 −7 | 1–10 | Melanin, black and green tattoos |
Picosecond alexandrite | 755 | Pulsed | 2 | 0.5 | 7.5 × 10 −10 | 1–10 | Melanin, black and green tattoos |
Diode (AlGaAs) | ~800 | CW/pulsed | 5–3000 | 5–100 | (5–400) × 10 −3 | 1–2 | Melanin, hemoglobins |
Nd:YAG | 1064 | CW | 10–100 | Hemoglobins | |||
Q-switched Nd:YAG | 1064 | Pulsed (ns) | 5–10 | 1 | 10 −8 | 5–10 | Black tattoos |
Long-pulsed Nd:YAG | 1064 | Pulsed | 1–50 | 1–50 | (1–200) × 10 −3 | 0–10 | Hemoglobins, melanin |
Picosecond Nd:YAG | 1064 * | Pulsed | 5 | 0.5 | 4 × 10 −10 | 1–10 | Melanin, black and red tattoos |
Long-pulsed Nd:YAG | 1320 | Pulsed | 1–50 | 1–50 | (1–200) × 10 −3 | 0–10 | Water |
Diode | 1450 | Pulsed | 10–15 | 10–15 | 0.15–0.25 | 1 | Water |
Erbium:glass | 1540 | Pulsed | 10 | 5 | 1 × 10 −3 | 2 | Water |
Holmium:YAG | 2000 | Pulsed | 5–100 | 0.2–5 | 10 −3 | 10–20 | Water |
Erbium:YAG | 2940 | Pulsed | 10–20 | 0.1–3 | (3–100) × 10 −4 | 5–100 | Water |
Carbon dioxide | 10 600 | CW/pulsed | 1–500 | 0.05–0.5 | 10 −5 –10 −3 | 10 2 –10 4 | Water |
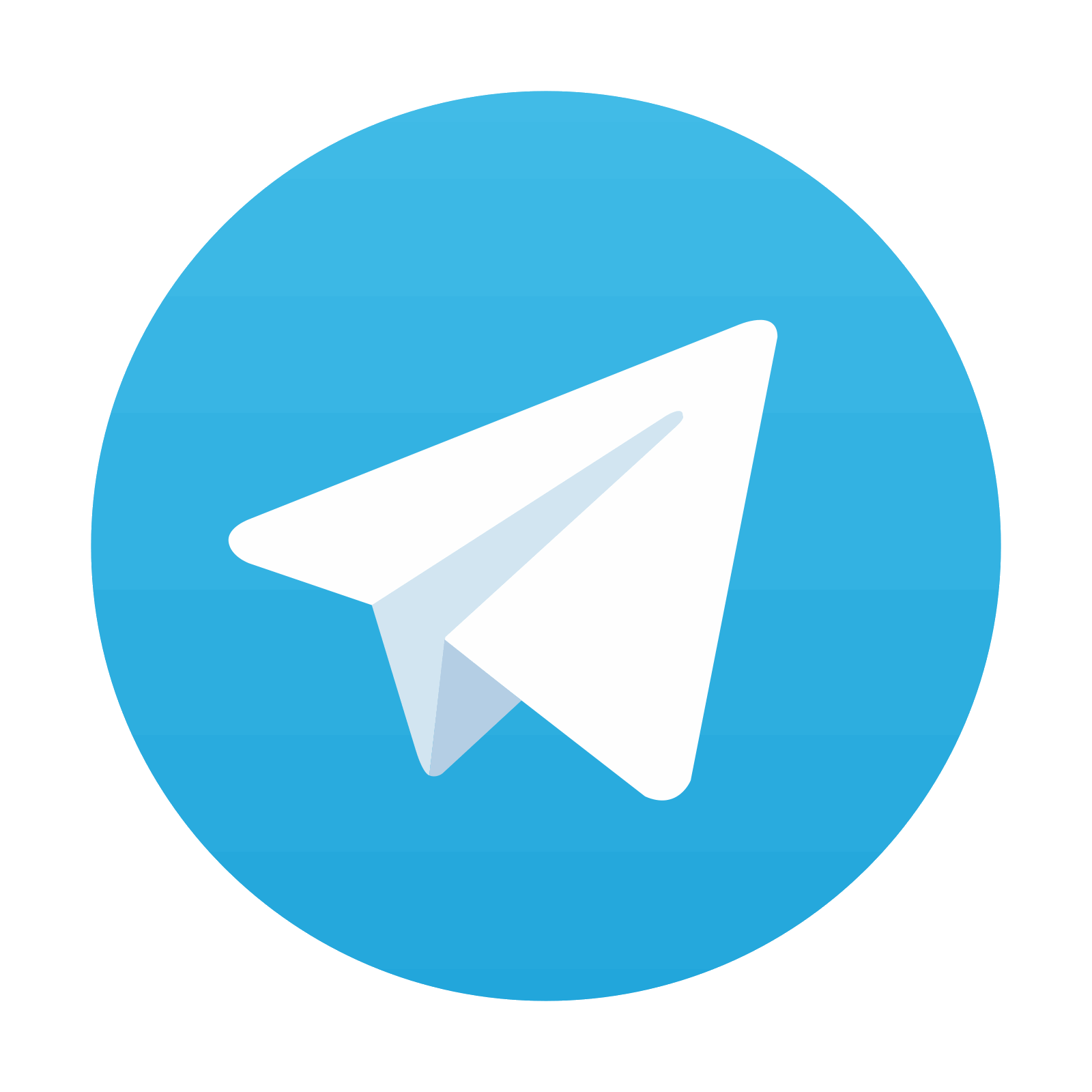
Stay updated, free articles. Join our Telegram channel
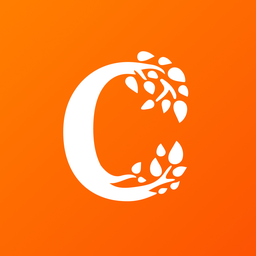
Full access? Get Clinical Tree
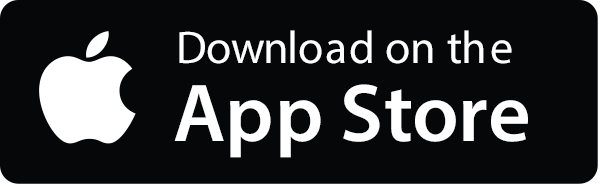
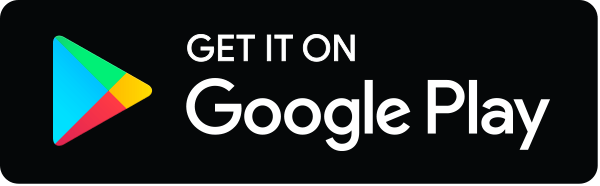
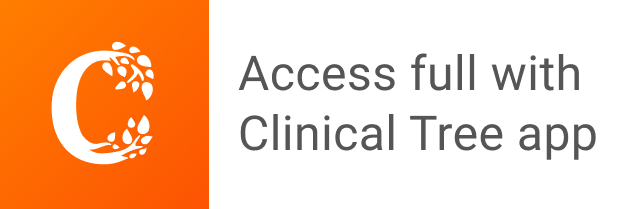