Fig. 2.1
Formation of “ice ball” during application of the timed spot freeze technique [5]. 1 Cryonecrosis area inside isotherm of −22 °C, 2 recovery area between 0° and −22 °C isotherms, 3 cutaneous surface, 4 epidermis, 5 dermis, 6 cryoprobe or cryospray, R lateral propagation of “ice ball,” and D depth of “ice ball.” In cryoprobe: D = 1.3 × R; in cryospray: D = 0.5 × R
Visual assessment of the cryogenic lesion (congelation) provides an opportunity to plan and manage the therapy.
Distinction is made between direct and indirect tissue damage mechanisms. Direct mechanisms involve both extracellular and intracellular formation of ice crystals as well as movement of water towards osmotic gradient. Also direct impact of cold on protein structure and enzyme systems is significant.
Indirect tissue damage mechanisms involve vascular stasis, ischaemia in the tissues and inflammatory and immunological responses. A summary of the events that occur during the cryosurgical procedure is given in Table 2.1 [5].
Table 2.1
The molecular basis of cryosurgical cell destruction
Molecular basis of cryosurgical injury | |||
---|---|---|---|
Mechanism | Time of cycle | Location | |
Direct injury | Extra- and intracellular ice crystal formation + coagulation necrosis | Freezing phase | Centre of the cryoinjury |
Vascular injury | Microcirculatory failure + ischemic necrosis | Thawing phase | Periphery of the cryoinjury |
Apoptosis | Cell death by apoptosis | Up to 8 h after rewarming | Periphery of the cryoinjury |
Immnunological | T-cell response mediatedby dendritic cells | Late event | Whole body |
2.3 Types of Heat Transfer
Rate of heat transfer determines the rate of tissue cooling. Rapid heat transfer occurs if there is a high temperature gap between cryogenic agent and tissues. This occurs when the temperature of a cryogen like liquid nitrogen (−196 °C) contacts the skin (36.6 °C).
Heat transfer basically occurs by two manners:
(a)
Boiling heat transfer: Cryogen comes in direct contact with tissues. Tissues lose their heat, while the cryogen heats up and evaporates. This is the process that occurs when spraying a cryogen on a tissue (as it occurs in cryospray freezing technique).
(b)
Conduction heat transfer: Through a direct contact (cryoprobe) with tissues, molecules of solid body transfer their kinetic energy to molecules of another body [4].
2.4 Cryogens and Cryosurgical Devices
2.4.1 Cryogens
Once a tissue has been subject to low temperatures, the achieved tissue temperature is the key factor in triggering tissue damage. A temperature of −20 °C in therapy of most neoplasms shall be cautiously evaluated as sufficient. Extensive damage of tissues occurs within the range of −20 and −30 °C, whereas destruction of cancer cells in this range is uncertain and incomplete. In vivo experiments show that vascular stasis after freezing-thawing has changed our understanding of lethal temperature in order to achieve therapeutic objectives. Neel et al. established the temperature necessary for destruction in experiments with animal tumours, t −60 °C, as well as argued for the necessity of repeated cooling, this was supported by later findings in studies of the topic [6–12]. In the experiments with diverse animal tissues, the range of lethal temperature is established between −40 and −50 °C [13–15]. Therefore, cryosurgery requires a cryogen capable of freezing at this temperature range. Some common cryogens are given in Table 2.2. Liquid nitrogen (LN) is the cryogen whose boiling point ensures reaching necessary parameters (Fig. 2.2) [3]. Necessary apparatuses are required to manipulate it.

Table 2.2
Temperature of some commonly used cryogens
Temperature of common cryogens | |
---|---|
Liquid nitrogen | −196 ºC |
Argon gas | −187 °C |
Nitrogen gas (N2O) | −88 ºC |
Carbon dioxide gas (CO2) | −78 ºC |
Organic compressed gases | −55 to −75 ºC |

Fig. 2.2
Boiling point of liquid nitrogen
2.4.2 Cryosurgical Devices
LN is commonly delivered to the skin by the following:
Dipstick (cotton swab) permeated with liquid nitrogen: This is a popular method where cotton swabs are dipped into liquid nitrogen and later transferred and contacted to the skin surface. This method prevents from reaching rapid cooling rate of tissues. This is a passive cooling technique in that, once the liquid nitrogen has evaporated, there is no new influx of cryogen, as happens in the case of cryoprobe or cryospray. The result is a noncontrolled freezing procedure. In addition, the surrounding liquid nitrogen may block vapour dissipation. It triggers the forming of an insulating layer which protects the site from pronounced cooling. This phenomenon is called the Leidenfrost effect. Therefore, a minimum heat transfer occurs between the dipstick and the skin, and cooling rate is low (<50 °C/min).
Not all cryogens and freezing devices allow achieving a sufficiently rapid cooling rate, whereas units operating without the liquid nitrogen and capable of achieving required cooling rates are too expensive.
Liquid nitrogen cryospray and cryoprobe: Liquid nitrogen comes into contact with the skin or metal of the cryoprobe on the surface of which rapid nitrogen evaporation occurs due to the heat transfer.
Cryospray and cryoprobe of liquid nitrogen allow achieving rapid cooling rate of tissues (>100 °C/min), because they allow for achieving high-temperature rates and because it is an active cooling technique.
Closed devices: A cryoprobe containing nitrous oxide operated based on the Joule–Thomson principle; namely, rapid expansion of the nitrous oxide occurs in closed space, which is an adiabatic process, and subsequently a quick drop of temperature of gas occurs. The aforementioned technique allows achieving only moderate cooling rates and is not available in a form of cryospray. Peltier thermoelectric modules (thermoelectric coolers/TEC) have similar deficiencies.
The use of pressurised gases as refrigerants (nitrous oxide, carbon dioxide and argon) spurred development of diverse instruments (thin needle-like probes, clamp devices, catheter probes and balloon structures), which in turn expanded indications of cryoenergy for treatment of a wide spectrum of diseases, including dermatologic, prostatic, hepatic, gynaecologic, ophthalmologic, neurosurgical and oncological disorders [16].
2.5 Monitoring of Cryogenic Lesion
Upon performing cryosurgical treatment of a skin lesion, the volume of frozen tissues can be monitored either visually or upon palpation. Frozen tissues visually become white towards the periphery from the point of cryogen incidence. When the afore-described ice ball is formed, there is interrelation between radius of the frozen region on the surface of the skin and depth of frozen tissues. If the cryoprobe is used, the depth of frozen tissues is 1.3 times greater than the radius on the surface of the skin, whereas in the case of cryospray, the depth is half of the radius [6].
Nowadays, instrumental methods are developed for monitoring freezing and volume of frozen tissues which allows reducing the risk of complications as well as ascertaining if sufficient degree of injury has been achieved. This is of utmost importance when dealing with malignant lesions. These instruments are as follows:
Thermocouple needles: It is possible to monitor tissue temperature using a thermocouple (thermoelectric element); however, in order to evaluate in-depth temperature, the skin has to be penetrated rendering it to be an invasive lesion-monitoring method.
Ultrasound: It allows real-time monitoring of the volume of frozen tissues and is easily available in outpatient practice as well. Cryosurgical equipment has also been developed where ultrasonic probes (transducers) are built in. Efficiency of the technique is limited by occurrence of acoustic shadowing [7].
2.6 Thermal Background of the Cryogenic Lesion and Variables
There are four parameters used to monitor depth, width and circumscription of cryogenic lesion. They are cooling rate, end temperature of tissues, hold time of frozen tissues and thawing rate [23].
2.6.1 Cooling Rate
Freezing rate of tissues may be fast (100–260 °C/min), moderate (10–100 °C/min) and slow (<10 °C/min) [6]. Since the objective is to destroy the tissues, reaching fast cooling rate is essential. In this case, intracellular crystal formation is accomplished inducing irreversible damage to the tissues and necrosis destroying cell organelles and membrane from the inside [23].
Slow and moderate cooling rates cause reversible damage to the tissues forming extracellular crystals, hyperosmolarity in extracellular space and dehydration of cells (Fig. 2.3) [24]. These processes induce programmed death or apoptosis in part of the cells [7]. It is important to note that cooling rates are higher in the center of the lesion, however closer to the periphery cooling rates are low to moderate [23].


Fig. 2.3
Extracellular ice formation leads to dehydration and shrinkage of the cells due to a high osmolality gradient. Rapid cooling rates allow intracellular ice formation that damages cell membranes. Subsequently, slow thawing leads to rehydration of the cells with their swelling or even bursting (Modified with permission from Erinjeri and Clark [24])
Modifications in cell damage according to the cooling rate are not directly proportionate. This is the so-called “inverted U” curve phenomenon (Fig. 2.4) [11]: cell death is marked at low freezing temperatures, when cells are dehydrating, and at high freezing rates, when intracellular crystals form. Between these parameters, high viability of the cells is also observed when cooling rate is sufficiently high to prevent dehydration; however, forming of intracellular crystals does not occur [11].


Fig. 2.4
Inverted “U” curve phenomenon: cell death is prominent at rapid cooling rates due to intracellular ice formation and at very slow cooling rates due to prominent solution effects (Used with permission from Takeda et al. [11])
2.6.2 End Temperature of Tissues
In order to accomplish tissue injury, a sufficiently low tissue temperature has to be reached. Tissue temperature determines the possibility of forming intracellular crystals by homogeneous nucleation, namely, spontaneous crystal nuclei formation inside the cells as long as the temperature is below −40 °C [25]. Furthermore, individual tissues and cells have different susceptibility to cold; thus, it is believed that lethal temperature of keratinocytes is at least −35 °C, while for endothelium it is −15 to −35 °C. On the other hand, upon treatment of benign lesions, the tissue temperature profile of at least −20 to −30 °C has to be reached, whereas in treating malicious lesions – at least −40 to −50°C [7] must be reached.
2.6.3 Hold Time of Frozen Tissues
Hold time of tissues in a frozen state enhances cryogenic injury mechanisms. Once intracellular crystals are achieved, recrystallisation will follow in time. As ice crystal grow in size, the more they will enhance cell injury. On the other hand, upon formation of isolated extracellular crystals, a more marked cell dehydration can be achieved [23]. It is believed that duration of freezing is not of critical importance, as long as the lowest tissue temperature reached is below −50 °C [7].
2.7 Vascular Cryogenic Injury
Under the impact of cold, delayed or indirect vasoconstriction of blood vessels occurs which induces occurrence of stasis and tissue ischaemia.
Damage to the endothelium of blood vessels during freezing and thawing is essential for cryolesion formation. Endothelium collapses when tissue temperature is in the range of −15 to −35 °C. The direct cell damage mechanisms described previously are crucial and include forming of crystals and cell dehydration [6, 23]. Nevertheless, it is also important to note that stasis caused by vasoconstriction facilitates hypoxia. Under the impact of hypoxia, cells release vasodilatation cytokines which after thawing enhance refractory vasodilatation and reperfusion injury which, among other, are triggered by excessive oxygen supply to the tissues and forming of free radicals. The free radicals induce lipid peroxidation in the membrane of endothelium cells. Reperfusion also facilitates inflammatory and perivascular oedema of tissues in the first 30 min already [26]. Intensified blood vessel permeability also contributes to occurrence of the oedema because perivascular tissues are dehydrated in the peripheral zone of the ice ball and shrink. That facilitates dilatation, stretching and increase of permeability [6, 23].
These processes create conditions which trigger blood clot formation according to Virchow’s triad: stasis caused by vasoconstriction, local thrombophilia triggered by reperfusion and exposing of connective tissue induced by endothelial damage. The first microthrombi appear within 2 h of treatment. Blood vessel clotting leads to ischaemia of tissues and subsequent coagulative necrosis which can be observed after 2 days [6].
2.8 Role of Inflammation and Apoptosis in Cryogenic Lesion
2.8.1 Inflammation
Inflammatory response develops within subsequent 24 h after cryosurgical treatment; it is a response to cellular death, especially in the centre of the lesion, where necrosis forms. Within 30 min of treatment, eosinophilic staining and vacuolisation of cell cytoplasm is observed, as well as nuclei becoming pyknotic, due to irreversible condensation of chromatin in the nucleus; epidermal-dermal separation occurs [4]. After vascular dilatation, oedema forms, which is especially marked in locations were the skin is loosely connected to deep tissues, for instance, dorsal surface of hands. Migration of cells to focal point of the lesion occurs, and neutrophils reach it first, whereas after 24 h macrophages and lymphocytes take over – by that time cell count in the lesion zone increases ten times (Fig. 2.5) [27]. In case of inflammation, pain occurs because kinins and substance P stimulates C fibre nociceptors [27]. Production of these agents is secondary to release of inflammatory cytokines in tissues, including TNF-α and IL-1 [27]. These cytokines facilitate production of eicosanoids (icosanoids). It should be noted though that freezing of nervous tissue itself may induce analgesia which will be described next.
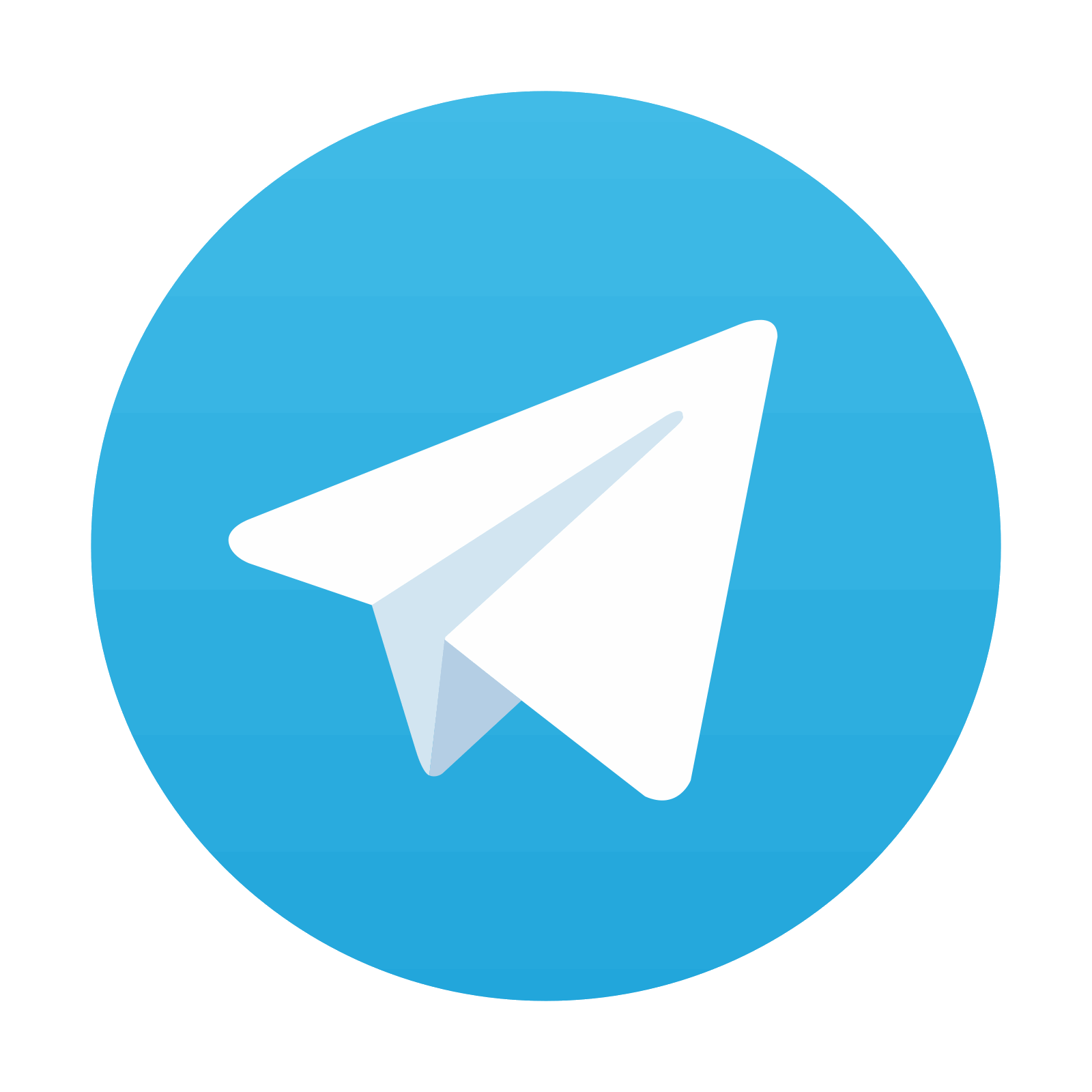
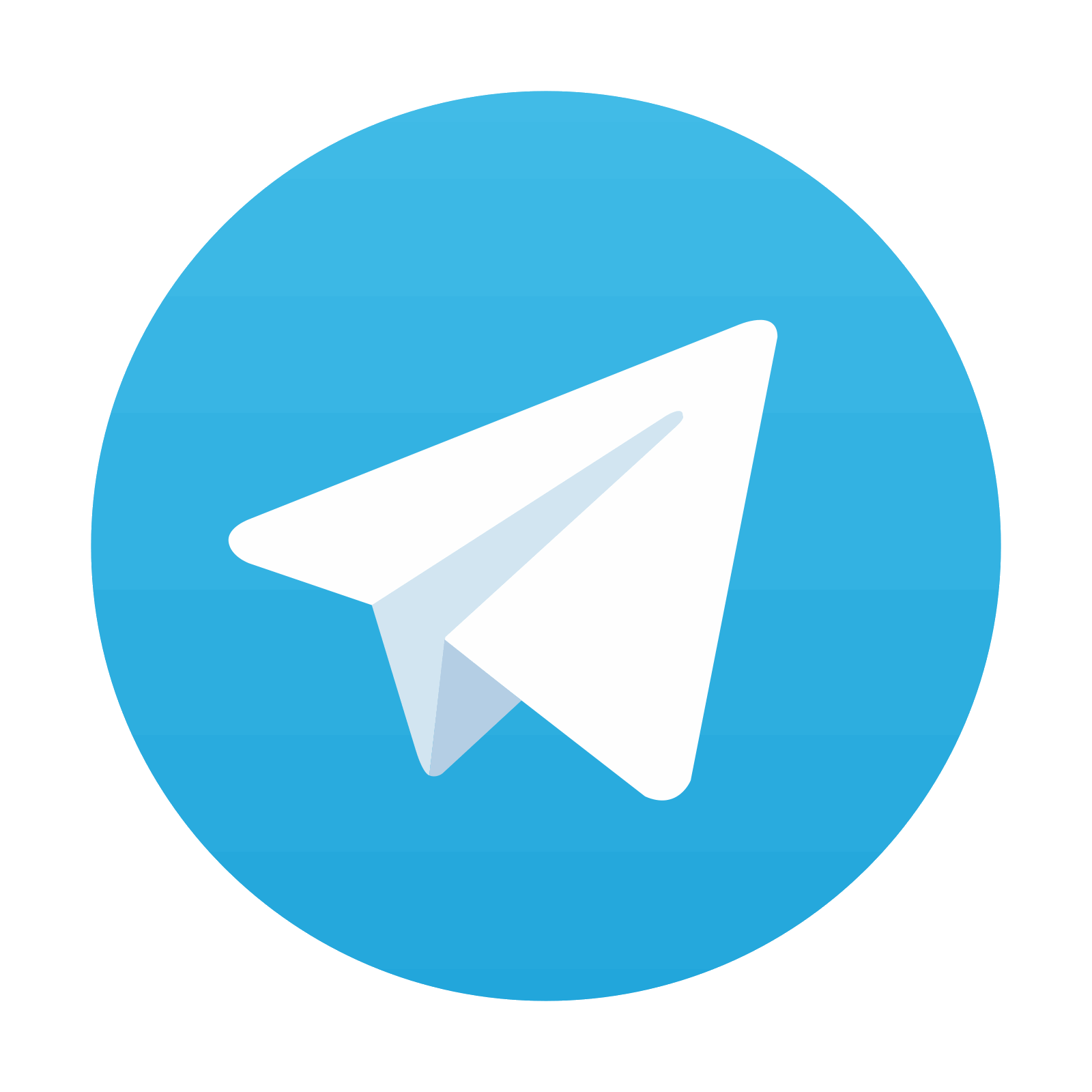
Stay updated, free articles. Join our Telegram channel
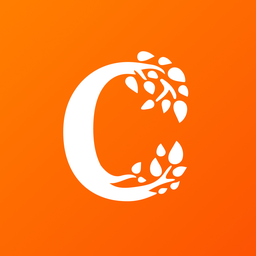
Full access? Get Clinical Tree
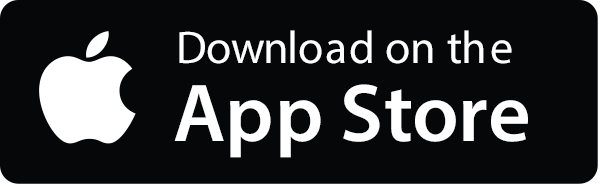
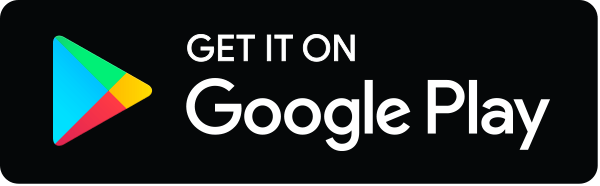