• Autologous bone and cartilage grafts remain important tissue sources for surgery of the facial skeleton due to their biocompatibility and long-term success rates.
• The revascularization of autologous grafts reduces long-term complications because the graft ultimately establishes a blood supply in its recipient site.
• The selection of different types of grafts is dictated by the functional needs of the defect.
• Mesenchymal stem cells can differentiate into osteoblasts and chondrocytes through upregulation of genes, such as Runx2 and Sox9, respectively.
• Revascularization of cancellous bone grafts occurs more rapidly compared with cortical bone grafts and correlates to the osteogenic potential of the graft.
• Cartilage is relatively avascular and has limited repair and regenerative capacity.
• Type I collagen is a key component of bone, whereas type II collagen is abundant in cartilage.
Introduction
Bone and cartilage abnormalities of the facial skeleton have a tremendous impact on facial aesthetics and may cause significant psychosocial burden to the patient. Anomalies of facial form may arise from acquired loss, congenital anomalies present at birth, or developmental anomalies that become evident with growth.
There are various grafting materials that can be used in skeletal facial surgery, including autologous tissues, allogeneic grafts, xenografts, or alloplastic implants. Autologous grafts will be the focus of this chapter. Autologous grafts remain the ideal option due to their limited immunogenicity, disease transmission, and risk of foreign body reaction and, thereby, higher success rates. The main disadvantages are the limited supply of autologous tissue, donor site morbidity, and variable resorption. Autologous grafts are useful tools in maxillofacial surgery. A thorough understanding of the biology of grafts will minimize complications and optimize results.
Although bone and cartilage both originate from a mesenchymal stem cell, their differentiation, proliferation, environmental niche, and reparative function differ significantly and will be discussed separately.
Bone biology
Composition of bone ( box 11.1 )
Bone, unlike other connective tissues, is a physiologically mineralized tissue. Bone is composed of three cell types, osteoblasts, osteocytes, and osteoclasts, as well as extracellular matrix (ECM). The ECM is composed of:
- 1.
Inorganic matrix, hydroxyapatite (50–70%)—the function of which is to strengthen the collagen composite, provide mechanical support, and serve as a source of calcium, phosphate, and magnesium.
- 2.
Organic matrix (20–40%)
- a.
Type I collagen—this predominant form is a triple-helical molecule composed of two α 1 (I) chains and one α 2 (I) chain; there are trace amounts of type III, V, and fibril-associated collagens.
- b.
Noncollagen proteins (NCPs)—coordinate cell–matrix or mineral–matrix interactions and regulate the mineralization process via osteoblast and osteoclast metabolism. There are four categories of NCPs: (1) proteoglycans, (2) glycosylated proteins, (3) glycosylated proteins with cell attachment activities, and (4) γ-carboxylated (gla) proteins.
- a.
- 3.
Water (5–10%)
- 4.
Lipids (<3%)
- 1.
Cells—osteoblasts, osteoclasts, osteocytes
- 2.
Inorganic matrix—hydroxyapatite
- 3.
Organic matrix
- a.
Type I collagen
- b.
Noncollagen proteins
- a.
- 4.
Water
- 5.
Lipids
The cell lineages that contribute to skeletal development include osteoblasts and osteoclasts. Terminally differentiated osteocytes also reside in bone, although they are thought to have limited regenerative capacity. Through complex cellular interactions and signaling molecules, these cells are responsible for the formation, growth, remodeling, and resorption of bone. Through the presence of mesenchymal stem cells, bones have the capacity for complete renewal, structurally and functionally. The mesenchymal stem cells allow for pluripotency, or the ability to differentiate into more than one cellular precursor ( Fig. 11.1 ). Those progenitors with upregulated Sox9 and Runx2 can differentiate into chondrocytes and osteoblasts, respectively, for endochondral or intramembranous bone formation.

Osteoblasts produce bone and can be further separated into cellular subsets based on their various genetic expressions. In early osteoblast maturation, type I collagen is upregulated, and this is later followed by expression of alkaline phosphatase and matrix gla protein. In the mineralization phase of osteoblasts, there is notable expression of osteopontin, osteocalcin, and collagenase. The bony ECM synthesis is partially regulated by C-type natriuretic peptide and the mitogen-activated protein kinase (MAPK) pathway of fibroblast growth factor signaling, whereas the NCPs (noted previously) are thought to play a central role in coordinating the cell–matrix interface, which can regulate osteoblast function. This complex network of cells and ECM works together to orchestrate bone formation.
Osteoclasts are responsible for resorption of the mineralized matrix, functioning to achieve optimal patterning and creating space for new bone formation during remodeling. Osteoclasts are unique because they are derived from the hematopoietic cell lineages, which are found in bone marrow, spleen, and peripheral blood. Osteoclasts have a common differentiation pathway with macrophages and have thus evolved distinct features, such as their multinucleated phenotype. Transcription factors, such as c-Fos, nuclear factor–kappa B (NF-κB), and microphthalmia-associated transcription factor, are necessary for the maturation of osteoclasts. In their functional state, osteoclasts secrete acids, collagenases, and proteases to resorb the mineralized matrix (osteoid) and associated proteins.
A critical balance between these two cell types is necessary to maintain bone. Receptors that mediate osteoclast formation are present on osteoblastic cells, indicating the importance of mesenchymal cell interaction in the recruitment of osteoclast progenitors. Receptor-activator of the NF-κB ligand (RANKL), a member of the tumor necrosis factor (TNF) superfamily, is located on osteoblasts and mesenchymal cells. Working together with its receptor RANK, found on osteoclast precursors, this interaction is essential for osteoclast activation and bone resorption.
Bone grafting
Free, nonvascularized bone grafts can be cortical, cancellous, or corticocancellous. Cortical, or compact, bone is the hard exterior providing the structure of bones and encasing the bone marrow space. Cancellous, spongy, or trabecular bone is less dense than cortical bone and is typically found at the ends of long bones, near joints, and within the iliac crest and vertebrae. The selection of the bone graft type will depend on the required function and mechanical load at the defect site; sites that require maintenance of structure or contour (onlay graft) or functional loading will require a cortical or corticocancellous graft (e.g., the orbital floor, mandible), whereas a site that requires bone augmentation with less functional loading can be constructed with a cancellous graft (alveolar cleft, an example of an inlay graft) regardless of the embryonic origin of the graft. , Additionally, grafts that are used for inlay augmentation tend to maintain or increase bone volume through remodeling, whereas onlay grafting resorbs over time, with the greatest resorption occurring with cancellous bone grafts.
In general, cortical bone grafts tend to resorb and remodel much slower. Autologous cortical bone grafts provide an osteoconductive medium with minimal osteoinductive and osteogenic properties. It is ideal for structural defects with immediate mechanical function, although adequate fixation and immobility are required for normal healing. The dense cortical matrix results in relatively slow revascularization and incorporation and limited perfusion and diminished viable donor osteocytes make this a poorly osteogenic graft type. In transplanted cortical grafts, revascularization must follow the Haversian system from the periphery to gain access to the interior bone ( Fig. 11.2 ). As a result, osteoclast resorption of necrotic donor trabeculae with osteoblast deposition of osteoid occurs, a process called creeping substitution. The new osteoid from the transplanted cells will later fuse to the native endosteum via consolidation. Differentiation within the graft is accomplished with osteoinduction from the BMPs found in the transplanted bone. Cortical grafts are incorporated by appositional bone growth, which may extend over months of remodeling; bone marrow may appear by 9 months following implantation and may be juxtaposed to resorbing necrotic bone islands. Counterintuitively, within the first 6 months after implantation, cortical grafts become progressively weaker due to resorption that outweighs bone formation; however, structural strength will improve within 12 months. During this phase, observation for mechanical failure is recommended, particularly in sites that are in a load-bearing region. Some examples of common harvest sites for cortical bone grafts include the iliac crest, cranium, and mandible (ramus). Corticocancellous sites of harvest include the iliac crest, rib, and mandible (symphysis). Each site should be considered according to the recipient site needs (size, shape, functional load), ease of retrieval, amount and type of bone needed, and potential risks to the patient.
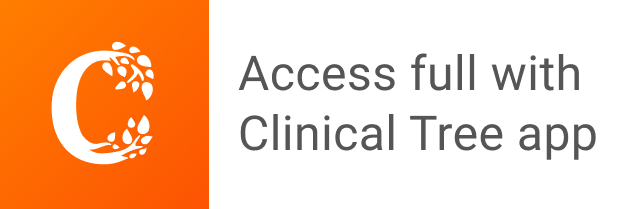