Key points
• The success of any implant depends not only on minimizing the inflammatory reaction of the material but also on the prosthetic porosity and stress at the tissue–implant interface.
• The porosity of an implant will dictate whether the implant becomes encapsulated (low porosity) or allows tissue in-growth (high porosity) leading to quiescence and toleration.
• Effective tissue repair with the use of allografts or synthetic implants should involve limiting the stress and strain at the implant– issue interface whether the implant is loaded in tension or compression.
• All nonvascularized implants induce a foreign body response as a result of macrophage activation.
• Calcium phosphate, hydroxyapatite, and ß-tricalcium phosphate are the most commonly used bioactive ceramics. They provide nucleation sites for deposition of an apatite layer and, if porous, allow for invasion of blood vessels.
• Most allografts do not come with the direction of the Langer lines marked; therefore, the surgeon does not know how to line up the collagen fiber orientation in the allograft with that of the host tissue (Langer lines). To minimize stress concentration at the interface, the Langer lines of the allograft need to be aligned with those of the host tissue.
Introduction
Alloplastic and natural materials have gained widespread use as replacements for tissues in a wide variety of applications. Allografts and xenografts have grown appreciably in their applications in a wide variety of clinical situations that range from the skeletal framework to the overlying soft tissue envelope (see Silver et al. for a review).
Synthetic materials, such as polyethylene terephthalate, polyethylene, and polytetrafluoroethylene, work well in many applications; metals, such as stainless steel, titanium, and cobalt chromium, are useful in stents; and natural materials, such as auto- and allografts, are used in many applications where cellular ingrowth and repair responses are required to promote long-term healing of tissues ( Table 12.1 ). Tissue responses to implants vary, depending on implantation site, material biocompatibility, implant porosity, and mechanical forces applied at the implant–tissue interface. Recent study results have suggested that mechanical mismatches at the tissue–implant interface can lead to implant failure due to upregulation of mechanotransduction processes.
CATEGORY | BIOMATERIAL | CLINICAL USE |
---|---|---|
Metals | Stainless steel | Mandibular joint prosthesis |
Cobalt–chromium | Fracture fixation plates | |
Titanium and alloys | Mandibular bone trays | |
Gold | Cranioplasty | |
Eyelid implants | ||
Osteointegrated implants | ||
Polymers | Polymethylmethacrylate | Cranioplasty |
Silicone | Solid implants | |
Polytetrafluoroethylene | Solid implants | |
Polyethylene | Solid implants | |
Polyurethanes | Wound dressings | |
Polyethylene terephthalate | Meshed implants | |
Polylactic/polyglycolic | Sutures/meshes | |
Ceramics | Bioglass | Ossicular replacements |
Hydroxyapatite | Joint components | |
Calcium phosphates | Bone replacement | |
Biologic materials | Collagen | Dermal augmentation |
Auto-allograft dermis | Skin repair | |
Auto-allograft bone | Cartilage repair | |
Demineralized bone | Bone augmentation | |
Growth factors | ||
Tissue-engineered skin | ||
Tissue-engineered cartilage | ||
Silk | ||
Chitosan | ||
Injectables | Hyaluronic acid | Filler materials |
Hydroxyapatite | ||
Polylactic acid | ||
Polymethylmethacrylate | ||
Autologous fat | ||
Engineered products | Tissue-engineered skin | Chronic skin wounds |
Tissue-engineered cartilage | Cartilage repair |
The purpose of this chapter is to discuss the use of synthetic and natural materials in aesthetic surgery of the facial skeleton. This chapter will also discuss biologic materials within the context of a broader body of scientific data. The success of any implant depends not only on minimizing the inflammatory reaction of the material but also on the prosthetic porosity and stress at the tissue–implant interface.
Effects of implant material characteristics, porosity, and interfacial stress
All foreign materials have limited biocompatibility. Any material without vasculature, whether it is a metal, polymer, ceramic, allograft, or autograft, is treated as a foreign body, triggering biologic cascades, including natural blood clotting, fibrinolysis, and activation of the complement and kinin systems. This leads to natural and acquired immune responses, including influx of inflammatory cells, release of cytokines and lymphokines, destruction of host tissue, and vasodilation. Thus the goal of the surgeon is to minimize these responses to prevent implant failure.
Beyond this, the porosity of an implant will dictate whether the implant is encapsulated or tissue ingrowth leads to movement of fibroblasts, capillaries, and stem cells into the implant, leading to quiescence and toleration. Reaching quiescence will depend on implant material characteristics and the host response—these factors will vary from patient to patient. If a thick capsule forms around the implant, the capsule will attempt to contract and may lead to shifting of the implant or to implant ejection from the host. In the case of porous implants, with pore sizes averaging greater than 100 to 150 μm, implants are stabilized by tissue ingrowth. However, the pores must be connected internally for appropriate tissue ingrowth.
In addition, there is strong evidence that the biomechanics of load transfer between native tissue and the implant plays a significant role in the functional success or failure of the implant. , Implants fail at the junction because of anatomic and biomechanical discontinuities. Effective tissue repair with the use of allografts or synthetic implants should involve limiting the stress and strain at the implant–issue interface whether the implant is loaded in tension or compression. In addition, the stress–strain behavior of tissues is nonlinear ( Fig. 12.1 ), with the modulus increasing according to the degree of deformation so that excessive strain at the implant–tissue interface magnifies the stress concentration at the boundary.

The testing of materials has moved from ex vivo to in vivo with increasing sophistication of the technology. The clinician today can measure the modulus of the implant, host tissue, and the interface to minimize implant-related problems and to develop better biologic materials by using optical coherence tomography (OCT) and vibrational analysis.
Adverse reactions to implants
All implants, with the possible exception of vascularized autograft flaps, have the potential to lead to adverse reactions. Adverse reactions can lead to intense pain, excessive inflammation, and even implant failure and extrusion. Implants initiate a macrophage-mediated acute inflammatory response, followed by resolution, proliferation of somatic cells, tissue remodeling, and tissue homeostasis. Macrophage polarization by cytokines leads to activation and then macrophage secretion of cytokines in the presence of an implant.
All nonvascularized implants induce a foreign body response as a result of macrophage activation. The sequence of events leading to this response includes (1) protein adsorption to the implant, (2) macrophage recruitment to the wound area, (3) macrophage adhesion to the implant surface, (4) release of macrophage chemokines, (5) recruitment of additional macrophages and immune cells, and (6) induction of acute inflammation.
Unresolved inflammation can lead to chronic inflammation that is accompanied by macrophage fusion into foreign body giant cells, followed by fibrous encapsulation of the implant. This can lead to movement of the implant and eventually implant failure. Proteins that are adsorbed to the implant and are involved in implant recognition include fibronectin, fibrinogen, vitronectin, and complement component C3b. Protein adsorption to foreign surfaces leads to initiation of a foreign body response.
Adverse reactions to metallic implants that contain nickel, cobalt, and chromium elements lead to cutaneous and systemic hypersensitivity. Adverse reactions to titanium, zirconium, cobalt–chromium, titanium–nickel, and steel have been reported.
Types of implant materials
A variety of implant materials, including polymers, metals, ceramics, and biologic tissues, have been used in surgery in the form of solid implants, porous materials, and injectables (see Table 12.1 ).
Metals
A number of metallic alloys, including stainless steel, cobalt–chromium, tantalum, titanium alloys, niobium, zirconium, and magnesium, are used in implants. Stainless steel was the first metal used in implants. The basic elements in stainless steel include iron, carbon, and other alloy elements, including chromium, nickel, and molybdenum. The most common type of stainless steel is 316 L, as designated by the American Society of Testing Materials (ASTM). This material has a lower strength, higher corrosion resistance, and higher stiffness compared with titanium and cobalt–chromium implants. Stainless steel is used for internal fixation devices because of its low price, easy availability, excellent fabrication properties, biocompatibility, and strength. Its negative attributes include pitting, crevice corrosion, and release of nickel and chromium. The corrosive properties of nickel are minimized by incorporation of chromium, molybdenum, and nitrogen.
In contrast, cobalt–chromium implants show high wear resistance and good mechanical properties; minimal tissue ingrowth and fibrous tissue formation around the implant are the negative factors. When metal-on-metal contact occurs, nanoparticles are created and promote the growth of common organisms. Nickel-free cobalt alloys are used in biomedical applications by using nitrogen as a substitute.
Titanium and its alloys demonstrate high strength, low density, high specific strength, good resistance to corrosion, inertness, moderate elastic modulus, and high rate of bone integration. , Titanium fiber meshes with 86% porosity and average pore size of 250 μm, and titanium foams have been constructed. These materials show characteristics resembling cancellous bone with high surface friction, improved porosity, and a relatively low modulus of elasticity. Titanium alloys, however, calcify when exposed to body fluids; these materials release aluminum and vanadium, and associated health problems have been reported.
Porous tantalum alloys are used for their ability to support bone ingrowth and their good cellular adherence and ingrowth with abundant extracellular matrix (ECM) deposition. , They are seen as a solution for their more active generation because they have adequate mechanical properties, are inert, and have excellent chemical stability.
Niobium and zirconium alloys show excellent corrosion resistance, fatigue strength, and crack propagation prevention. They belong to the same elemental groups as titanium but have improved properties. Ceramification of zirconium and niobium alloys leads to improved wear properties.
Magnesium alloys possess mechanical properties very similar to those of bone; with magnesium being the fourth most abundant element in the human body, its degradation products can be recycled or excreted through normal pathways. Magnesium alloys exhibit less corrosion resistance compared with titanium and cobalt–chromium alloys, so modifications are needed to improve corrosion resistance and to slow down resorption.
The use of alloplastic materials in cranioplasty has recently been reviewed. Gold, silver, platinum, aluminum, and titanium have all been used historically. Titanium alloys are often used as a secondary source of repair materials after autologous bone repair has failed.
Ceramics
Both inert and bioactive ceramics are used as implant materials. Inert ceramics include alumina and zirconia, which demonstrate excellent corrosion resistance, biocompatibility, low frictional coefficient, high wear resistance, and stability. In applications with low mechanical loads, low fracture rates are observed. Improved crack resistance is observed in zirconia–alumina composites.
Bioactive ceramics include calcium phosphates, glass, and glass–ceramic mixtures and composites. Calcium phosphates show excellent biocompatibility, bioactivity, and biodegradability and are osteoinductive. Calcium phosphate, hydroxyapatite, and β-tricalcium phosphate are the most commonly used bioactive ceramics. They provide nucleation sites for deposition of an apatite layer and, if porous, allow for invasion of blood vessels.
Glass-based scaffolds include glass/glass ceramic scaffolds and glass–polymer composites. Elemental silicon found in these materials upregulates gene expression of osteoblasts and production of growth factors.
Polymers
A number of synthetic polymers are used in medical implants (see Table 12.1 ). They include acrylics, polyamines, silicones, and ultra-high-molecular-weight polyethylene. Polymethylmethacrylate (PMMA), due its elastic modulus being similar to that of bone, is used in many applications. Polyhydroxyethylmethacrylate and polymers containing hydroxyapatite have osteoconductive properties. However, in some applications, these polymers lack adequate mechanical stability; fiber-reinforced composites are needed for these applications. Natural polymers, including collagen, silk, and chitosan, have received recent attention as replacement materials.
Autografts, allografts, and xenografts
Recent studies have reviewed the use of autografts in facial surgery. , Synthetic materials, such as Dacron and Teflon, work well in many applications; stainless steel and other metal wires are useful in stents; and natural materials, such as auto-, xeno-, and allografts are useful in many applications where cellular ingrowth and repair responses are required to promote long-term healing of tissues, such as the dermis and ligaments. Although many studies have underscored the utility of dermal allografts, questions arise regarding the effects of processing conditions, such as decellularization, decontamination, and viral deactivation, on the structure of these tissues and the surgical outcome. More than one third of hernia repairs require revision surgery due to mechanical mismatches at the implant–host tissue interface. Therefore the mechanical properties of grafts appear to be important characteristics needed for a positive outcome, at least in hernia repair.
The primary component of allograft tissue is the ECM, which is composed of collagen and elastic fibers, proteoglycans, cell attachment factors, growth factors, signaling molecules, cells, ions, and water. The three-dimensional (3D) structure of collagen scaffolds has been shown to promote the repair response in dermal and orthopedic applications by minimizing the deposition of granulation tissue and promoting organized collagen deposition along the major axis of the scaffold. The results of a recent study have suggested that the decellularization process of allografts leads to collagen network swelling and increased stiffness of the dermis. This may lead to modulus mismatches when allografts are sutured to host tissue.
Moore et al. reported the effects of using a nondenaturing anionic detergent, recombinant endonuclease, and antibiotics on the decellularization and mechanical properties of the human dermis. They showed that 97% of the DNA was removed, as well as cell membrane components, such as major histocompatibility complex-I, yielding materials with ultimate loads of up to 635 newtons (N). Terzini et al. reported decellularization in 0.06 N sodium hydroxide (NaOH) for 1 to 7 weeks at room temperature. They found that the ultimate stress of the product was between 4 and 10 megapascals (MPa) with 5 to 6 weeks of treatment and that longer treatments reduced the product’s strength. It is important to understand how processing conditions affect the physical structure and properties of decellularized allografts.
Injectable implants
The history of injectable materials is long and complex. Facial fillers used historically include autologous fat, paraffin, injectable silicone, Teflon, bovine collagen, and hyaluronic acid. Adverse reactions to many of these products have led to the introduction of a new generation of materials, including microspheres of hyaluronic acid, calcium hydroxyapatite, and PMMA, formulated to enhance longevity (see Table 12.1 ).
Mechanical properties of implants
Beyond the need to characterize mammalian tissues by mechanical means, it is important to be able to characterize implants that are used as replacements or to augment tissues. The majority of polymers used in medicine are polyglycolic acid, polylactic acid, polydimethylsiloxane, polytetrafluoroethylene, polyethylene, polyacrylates, polyurethanes, and natural polymers, such as collagen, hyaluronan, alginates, and silk (see Table 12.1 ). Their properties vary extensively, depending on how they are compounded and processed. Clearly, the strength and stiffness of synthetic sutures, wound dressing materials, bandages, and grafts are important parameters that need to be understood to prevent premature mechanical failure of medical devices.
The mechanical properties of implants and tissues, both in vitro and in vivo, have been the subject of extensive research and books. Most mechanical tests require the use of stresses and strains that cause permanent damage to tissues and implants. Ultrasonic and elastographic measurements do not destroy tissue, but these measurements require the use of models for tissue behavior that assume linear Hookean behavior (stress and strain are linearly related) and incompressibility (Poisson’s ratio of 0.5)—two assumptions that lead to incorrect determinations of mechanical parameters.
Mechanical properties of materials
For the simplest loading condition in a single direction of an ideal material, stress (force per unit area) and strain (the change in length divided by the original length) are linearly related through a constant termed modulus or stiffness (E), as indicated in the equation below:
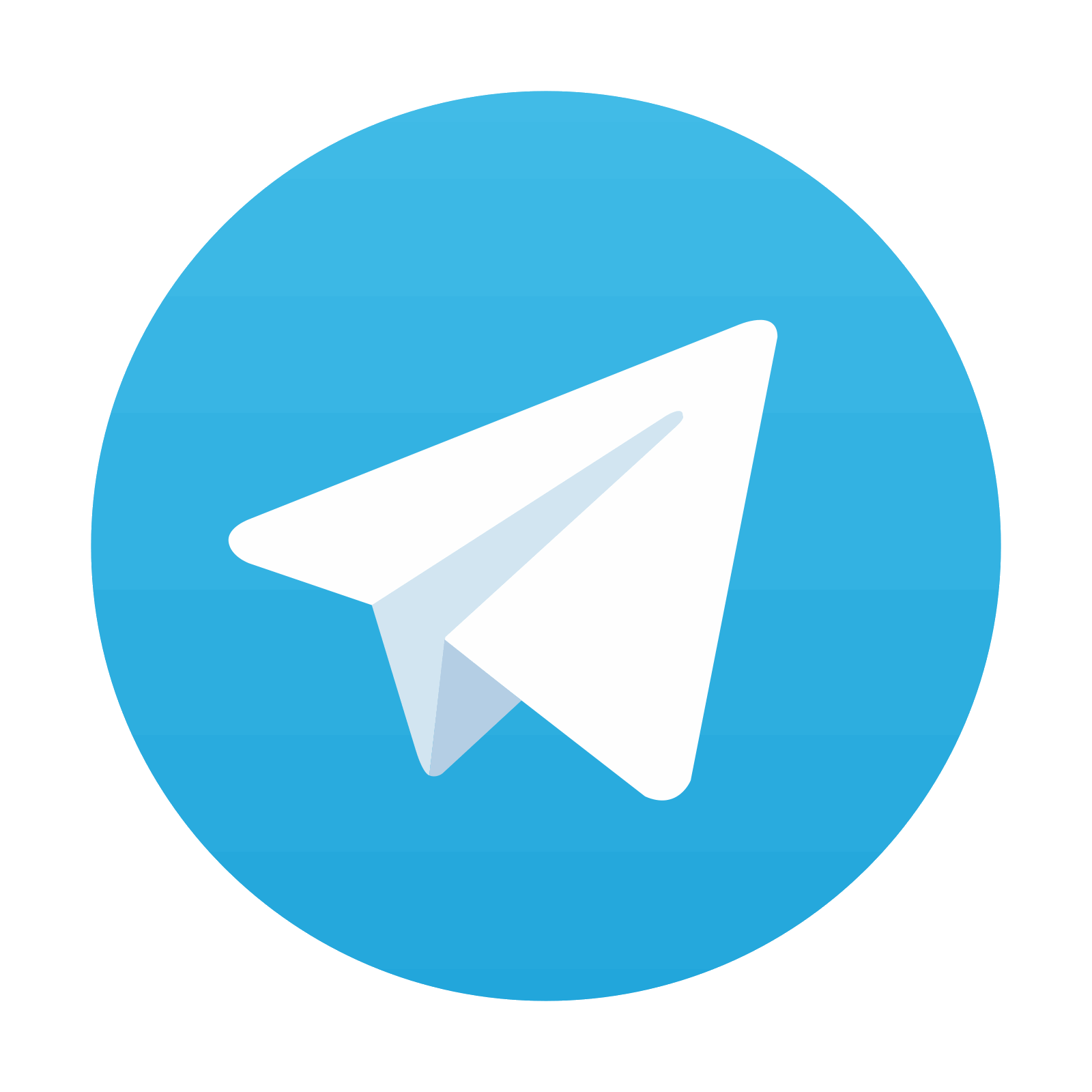
Stay updated, free articles. Join our Telegram channel
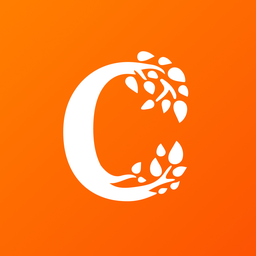
Full access? Get Clinical Tree
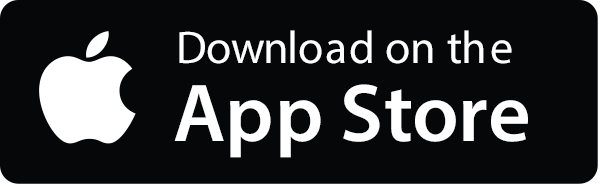
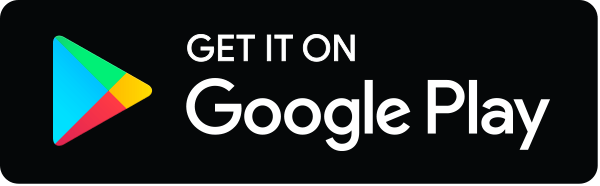
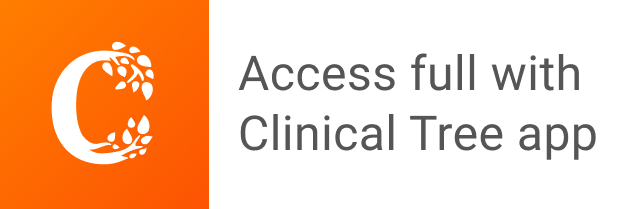