Fig. 14.1
Differentiation of ADSCs into several ectoderm, mesoderm, and endoderm lineages
The SVF population includes cells contained within the stroma (interstitial cells such as fibroblasts, preadipocytes, and tissue macrophages) and vasculature (endothelial cells, vascular smooth muscle cells, and pericytes) of adipose tissue. In essence, this population includes all cells present within the adipose tissue, with the exception of lipid-laden adipocytes. To better characterize the composition of SVF, a combination of cell surface markers are used to identify the various cell populations within SVF. As published by multiple groups [12–18], freshly isolated SVF contains a heterogenic population of blood-derived cells (CD45+), adipose-derived stem cells (ADSC)/fibroblast (CD31−CD34 + CD45−CD90+), endothelial (progenitor) cells (CD31 + CD34 + CD45−), pericytes (CD90 + CD146+), and other cell populations. On the other hand, with progressive culturing and passaging, SVF becomes a more homogenous mesenchymal stromal cell population (ASCs) based on its plastic adherent properties [12–18]. In general, the ASCs population loses the expression of CD45+ andCD34+ and increases the expression level of CD73+, CD90+, CD105+, and CD44.
14.2 SVF Isolation
SVF cells are obtained by first washing the adipose tissue to remove blood cells, which are an inevitable contaminant of the liposuction process, followed by treating the washed tissue with collagenase to release the cells from the extracellular matrix. Adipocytes are then removed on the basis of their natural buoyancy, leaving behind an adipocyte-depleted population of stromal and vascular cells [1, 19].
The enzymatic approach yields a large number of viable nucleated cells and has been considered the gold standard means for obtaining these cells for almost 50 years [20]. Recently however, nonenzymatic approaches such as emulsifying agents and ultrasonic energy have been suggested as alternate means for extracting SVF from adipose tissue. The composition of the cell population generated by newly proposed methods to that obtained with the gold standard enzymatic method was recently compared [21]. The data shows that the viable nucleated cell yield obtained with the emulsifier was 10 % that of the enzyme-derived SVF (Fig. 14.2). Upon checking the population under the microscope, it appears the cells are primarily blood cells (Fig. 14.3). Importantly, when the population was examined using the standard assay for stem cells (the CFU-F assay), no adherent colonies were detected. By contrast, the CFU-F frequency in the enzymatically isolated population obtained from the same tissue ranged from 0.5 to 2 % of the plated cells.
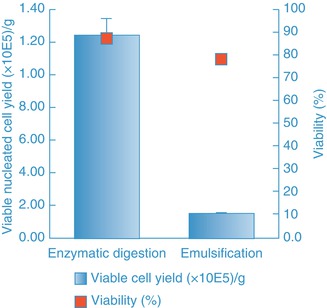
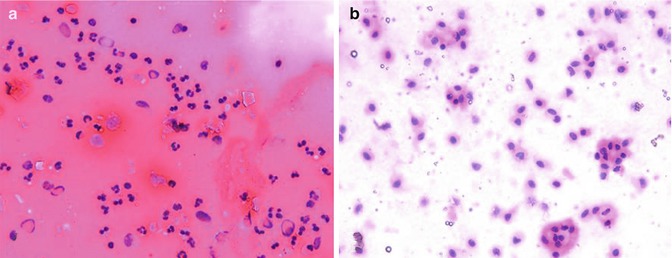
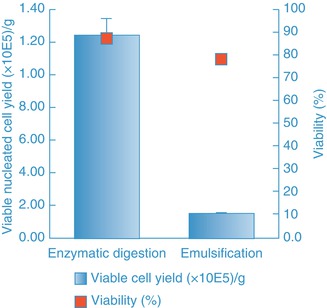
Fig. 14.2
Viable nucleated cell yield and viability of cell populations generated by either an emulsification method or enzymatic (in an automated system, Celution®) digestion method
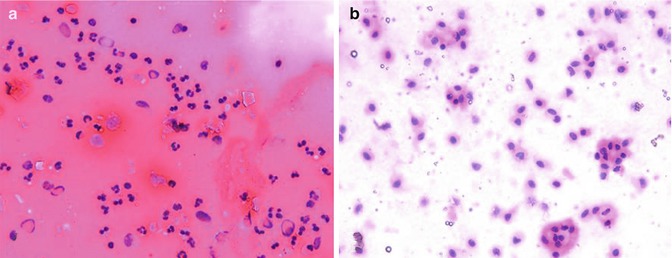
Fig. 14.3
Cytological morphology evaluation. (a) Emulsified sample contains a dense concentration of red blood cells. The bi- and trilobar morphology of the majority of cell nuclei is indicative of a neutrophil/basophil phenotype. (b) SVFs from enzymatic digestion plated at the same nucleated cell density contain far fewer red blood cells. The large uni-lobar nuclei are typical of vascular endothelia, pericytes, monocyte/macrophages, and adipose-derived stem cells
Evaluation of ultrasonic energy at various power and amplitude settings, as well as with different sized sonotrodes, showed similar results. Specifically, low (33 % duty cycle, 35 % amplitude) and medium (33 % duty cycle and 55 % amplitude) energy settings led to a cell yield that was no greater than controls in which the device was not powered on (Fig. 14.4) and like the emulsifier was comprised primarily of blood cells. CFU-F and CD34-positive cell content in samples processed with ultrasound energy was considerably lower than that obtained with enzymatic digestion (Fig. 14.5). At higher energy settings, ultrasonic energy destroyed adipose tissue and yielded mainly cell debris. In summary, these alternative processing methods yield a population that is comprised primarily of blood cells rather than stromal and vascular cells, and consequently, the output population from such methods is not SVF.
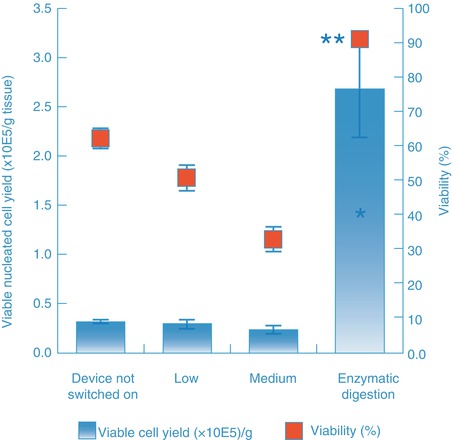
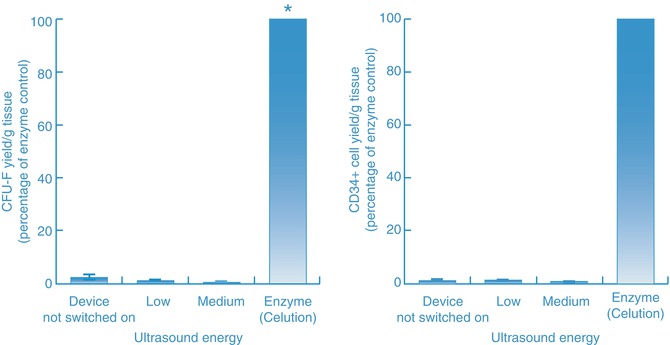
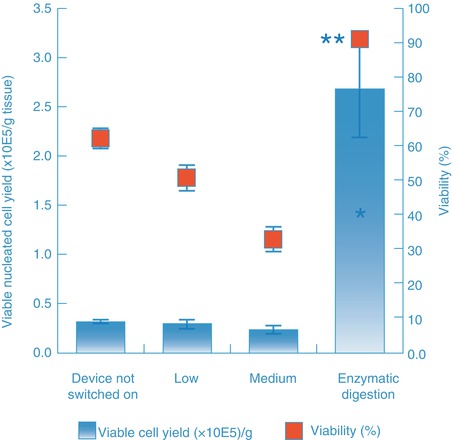
Fig. 14.4
Viable cell yield and viability of cell populations generated by either the ultrasonic cavitation method or the enzymatic (in an automated system, Celution®) digestion method. *Show significance of viable nucleated cell yield at P < 0.05 level, when compared to each ultrasonic treated groups using paired t test, N = 5. **Show significance of cell viability at P < 0.001 level, when compared to each ultrasonic cavitation groups
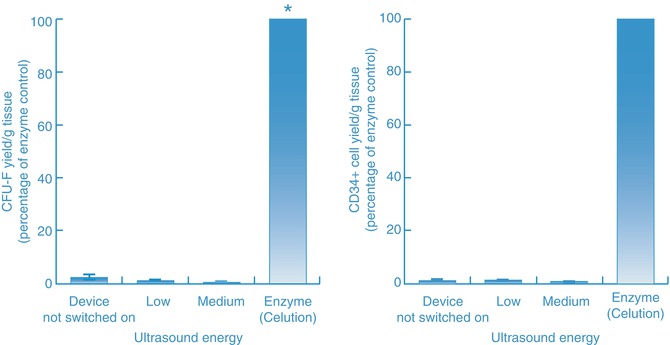
Fig. 14.5
Stem cell (CFU-F) yield and CD34-positive cell yield of cell populations generated by either the ultrasonic cavitation method or the enzymatic (in an automated system, Celution®) method. Enzymatic processing yield between a 14.5- and 22.2-fold increase in CD34+ cells compared to samples treated with ultrasonic energy (at low and medium setting) and exhibited a CFU-F frequency that was 600 % greater than the low energy setting and 1,500 % greater than the medium energy setting
The findings support the retention of enzyme-based method as the gold standard to obtain SVF cells from adipose tissue. This should not be surprising, in that the integrity of the extracellular matrix that binds individual adipocytes into clusters and links vascular cells into a strong leak-resistant structure is a biologic necessity. Collagen, the key component of this matrix, is comprised of three protein chains coiled into a triple helix and cross-linked into a single entity. Multiple triple helices assemble in staggered form into a collagen fibril, and multiple fibrils in turn assemble into a collagen fiber. Collagenase has evolved over millennia as a specific and highly efficient method of degrading collagen, to allow for tissue remodeling and repair (e.g., human metalloproteinases) or pathology (e.g., bacterial collagenase). As a result, during enzymatic processing the cells are released from their niches in an efficient, specific, and gentle fashion, compared to physical methods that apply energy in nonspecific fashion to the whole tissue. Furthermore, the data shown above emphasizes the need to evaluate outputs obtained by alternate processing methods, using robust characterization that includes description of the composition of the cell population, instead of simply the number of cells or particles extracted. In particular, methods that purport to isolate this population must indeed be shown to generate a population that is comprised predominantly of stromal and vascular cells.
14.3 Potential Regenerative Mechanism of SVF
The observation that ADSCs can differentiate into multiple cell lineages suggests that the differentiation may be an important mechanism by which the cultured cell population would provide benefit. By extension, this argument might also be applied to the SVF population from which these cultures are derived, particularly given the high frequency of stem cells (CFU-F) in SVF [22]. However, an increasing body of evidence has shown that in vivo differentiation contributes little, if anything, to efficacy seen in preclinical and clinical application of either ADSCs or SVF. Rather, efficacy appears to arise through the ability of the SVF population to deliver multiple growth factors in a paracrine fashion and thereby promote tissue healing and regeneration [23, 24].
14.3.1 Angiogenesis
SVF contains vessel-forming cells including endothelial, smooth muscle, endothelial progenitor cells (EPCs), and pericytes. In addition, SVF cells secrete numerous angiogenic growth factors including bFGF, PDGF, PlGF, VEGF, and HGF [9, 25, 26]. Angiogenesis is a key factor for successful incorporation of an autologous fat graft into the recipient site and for long-term retention of the graft [9, 27–29]. The ability of SVF cells to promote angiogenesis may explain why supplementation of fat grafts with these cells leads to a significant increase in graft retention [9, 30].
14.3.2 Anti-apoptosis
The SVF population also has the potential to reduce cell apoptosis by means of growth factor secretion and direct cell-cell interaction. Both Wang et al. and Zhu et al. have demonstrated that growth factors VEGF, HGF, and IGF-1 are secreted by SVF [9, 31]. VEGF and HGF are known to act in a mutually complementary way to reduce cell apoptosis [32, 33], while IGF-1 can rescue cells from apoptosis through activation of the PI3-kinase pathway [34]. Moreover, it has been shown that direct cell-cell contact with macrophages rescue damaged myocytes from apoptosis by decreasing annexin V activity and caspase-3 activity and increasing Bcl-2 survival signal within the damaged cells [35]. With the anti-apoptosis mechanism, SVF can rescue graft tissue from hypoxic conditions and thus limit the extent of graft tissue damage and apoptosis.
14.3.3 Anti-inflammation and Anti-scar Formation
Another potential therapeutic role of SVF is its ability to decrease local inflammation and reduce further tissue damage caused by activated leukocytes. Extensive infiltration of activated leukocytes may cause tissue remodeling pathogenesis, as well as promote cell and tissue apoptosis [8, 36]. Recent in vitro and in vivo studies indicate SVF reduce leukocyte (CD3+) infiltration; increase expression of anti-inflammatory factors such as IL-10, prostaglandins, INF-gamma, and HGF; and reduce expression of proinflammatory cytokines such as TNF-alpha, IL-6, and TIMP-1 [8, 36]. Studies have shown that SVF-mediated reduction in inflammation is associated with a reduction in the size of the scar [8]. This mechanism may explain preclinical data showing that supplementing fat grafts with SVF cells leads to reduced tissue fibrosis at both 6- and 9-month follow-up time [9].
14.4 SVF as Fillers in Plastic and Reconstructive Surgery
The ability to move fat from one location into another has long been a desire of plastic surgeons. There are numerous applications in both cosmetic and reconstructive settings, where autologous fat transfer would be extremely beneficial. Fat grafting (AFG) has been used to treat patients with volume loss due to disease, trauma, congenital defects, or the natural process of aging [37]. To date, however, the predictability and survival of grafted adipose tissue has been poor [38]. As described in several review papers [39–41], the disparate reports of clinical success for AFG, particularly for large-volume indications, appear to be a result of both technical variability among physicians and the biology of the tissue itself. In retrospect, an obvious correlation exists between autograft volume and transplant efficacy. When graft size exceeds the volume through which oxygen and nutrients can passively diffuse, long-term graft retention rate is dramatically reduced as a result of cell death from tissue ischemia. Subsequent, tissue inflammation and extracellular matrix remodeling may further reduce the amount of remaining healthy tissue.
This problem has been addressed by the development of various graft tissue harvesting, preparation, and delivery methods. It is widely accepted that using low-pressure harvesting techniques and removing nonviable, proinflammatory components of lipoaspirate (including oil, blood, damaged tissue, and debris prior) prior to reimplantation will result in a pure, viable graft tissue and thus lead to superior long-term graft survival [39–41]. Similarly, using a graft delivery method that deposits the graft material in small parcels, and injecting through multiple access sites into various soft-tissue depths, allows grafted tissue to be more amenable to receiving nutrients and oxygen passively [39, 40]. Nevertheless, even with improved delivery technique, there remains a graft volume beyond which graft retention is compromised. The precise volume limit will likely be unique to each patient and be particularly low for patients in whom the graft bed is not well vascularized or has chronic inflammatory, scarring, or prior radiation exposure. The angiogenic, anti-apoptotic, and other properties of SVF discussed above provide a potential solution for large size grafting or patients with complicated conditions. Because SVF cells are embedded throughout the fat graft, there are relatively few at the surface of the graft. This is important, because the signals released at the surface of the graft from SVF can stimulate angiogenic sprouting from nearby vascularized tissue. The principle behind cell-enriched fat grafting is that SVF cells extracted from a second aliquot of tissue can be used to coat the graft with extra SVF cells, thereby dramatically increasing the number of growth factor-producing cells at this critical interface.
In one study, the number of cells coating SVF-enriched graft and the number coating grafts prepared by centrifugation were compared. The results show that the cell-enriched graft contained 10-fold more nucleated cells, 104-fold more endothelial cells, and 62-fold more preadipocytes than grafts produced by centrifugation [42]. This data, combined with the enhanced retention observed with cell-enriched grafts seen in controlled preclinical studies, strongly suggest that the angiogenesis, anti-apoptosis, anti-inflammation, anti-scar formation, and tissue remodeling properties of SVF are a key reason for the increased graft retention.
In a side-by-side clinical study reported by Sterodimas’ group [43], patients with congenital or acquired facial tissue defects were treated. The patients were randomized into two groups with ten patients in each group. Patients who received cell-enriched grafts reached targets for both patient and physician satisfaction with only a single transplantation procedure. At 6 months follow-up, significantly better graft retention was reported with the group of patients who received cell-enriched graft transplantation. By 18 months, however, the significance between two groups disappeared. It needs to be taken into consideration that seven of ten patients with graft-only treatment received more than one AFG procedures.
Under the same premise, Yoshimura et al. applied the cell-assisted lipoaspirate (CAL) technique for treatment of facial lipoatrophy, cosmetic breast augmentation, and breast implant complication rescue [10, 24, 44]. Both Yoshimura and Matsumoto observed that cell-assisted lipotransfer promoted graft retention rates [24, 30], and only 2–3 % of patients presented minor complications such as cyst formation or micro-calcifications.
With increased attention given to SVF-assisted fat grafting tools, it is necessary to consider the safety of this new technology. Cultured bone marrow mesenchymal stem cells (MSCs) have been shown to enhance in vivo metastasis of breast cancer cell lines [45]. ADSCs have also been shown to increase in vivo growth of a murine mammary cancer cell line [46] and migration of a human breast cancer cell line [47]. Others have reported that this effect is limited to stimulation of active, but not resting, tumor cells from clinical breast cancer samples [48]. These studies, however, need to be interpreted within the context of clinical relevance, rather than under the laboratory conditions in which they were performed. First, they were executed with cultured ADSCs, not with freshly isolated SVF cells. Secondly, in the study performed to analyze MSC-induced metastasis, it was shown that this phenomenon required that the MSCs and tumor cells be mixed and implanted together in order for metastasis to increase; placing the two cell types nearby one another was insufficient to increase metastasis [45]. Follow-up mechanistic studies similarly demonstrated that cell-cell contact was required to initiate the metastatic process [45]. This level of cell-cell contact is extremely unlikely in the context of a soft-tissue defect repair with the SVF-enriched graft. It should also be noted that other groups report that ADSCs exhibit a tumor-suppressive effect [49, 50]. It is also possible that expression of pro-angiogenic growth factors by SVF cells might increase tumor formation or recurrence; however, analysis of human breast cancer shows high levels of expression of angiogenic factors by the majority of breast tumors and limited efficacy of anti-angiogenic agents [51, 52]. These observations indicate that breast cancer growth is not limited by extra-tumoral pro-angiogenic factors. The cumulative result of this evidence, combined with clinical evidence indicating the safety of autologous fat grafting in the breast [42, 53–55], suggests that the risk, if any, is extremely limited, though as with any new technique, appropriate monitoring and follow-up should be performed.
According to Gir’s [53] recent review, there are 174 published cases and five registered clinical trials that have been published in plastic and reconstructive clinical applications using autologous SVF, either by itself or in combination with fat grafting. Doctors in Brazil, Europe Japan, Korea, and the United States have applied SVF to treat patients with lipodystrophy, Parry-Romberg disease, and depressed scars, as well as to reconstruct breast deformities. The results thus far are encouraging in both large-volume and complicated soft-tissue filling application, as well as in challenging wound healing indications [53].
14.5 Automated Isolation of ADRCs or SVF for Clinical Applications
Enzymatic digestion is the gold standard for isolation of SVF from adipose tissue. SVF yield (viable nucleated cell number/g tissue), composition, and differentiation potential when placed in culture can be affected by donor age, gender, race, donor site, health condition, as well as adipose tissue harvesting technique [56–58]. However, variables such as the amount of extravascular blood present in the tissue prior to digestion; the composition, source, and concentration of the enzyme used in the isolation procedure; digestion temperature; duration of digestion; and residual enzyme level in the final output also result in variability in the composition of the product obtained after processing. These variables can be controlled by application of an automated device that provides a standardized processing procedure.
There are several devices available on the market that claim to automatically isolate SVF from adipose tissue in real time. These include Cha-station/Multi-station (P&C International, Korea), Sepax® system (Biosafe Group SA, Switzerland) [59], Lipokit/Maxstem (YC-100, Medi-Khan, Korea), HuriCell (Lyle Services Corp, Korea), IcellatorTM Cell Isolation System (Tissue Genesis, Honolulu, HI, USA) [60], and Celution® System (Cytori Therapeutics, Inc, San Diego, CA, USA) [13]. None of the devices have yet been cleared or approved by the US FDA for commercial distribution for clinical applications. Most importantly, in evaluating various devices, it is critical that the composition of the output, in terms of both cellular and noncellular (residual enzyme, endotoxin levels, etc.) elements, is thoroughly characterized. As noted above, simple reporting of viable cell counts is inadequate. Systems designed for counting particles or blood cells can lead to enormous overestimation of cell yield. Furthermore, a cell product should not be referred to as SVF, irrespective of cell yield, if the population is not comprised predominantly of stromal and vascular cell types. Noncellular constituents such as extracellular matrix components and residual enzyme must also be considered, as they can lead to substantial risk for patients, particularly following intravascular or intradermal administration.
Until now, only the Celution® and IcellatorTM Systems have been approved by the FDA to initiate clinical trials. Cytori is currently using the Celution® System to conduct the ATHENA trial, a safety and feasibility study on patients with chronic ischemic heart disease, and Tissue Genesis is using their IcellatorTM System in a bypass grafting feasibility study to evaluate adipose-derived cell coatings on ePTFE vascular grafts.
The term adipose-derived regenerative cells, or ADRCs, describes SVF that has been found to meet appropriate safety and sterility criteria and as such is suitable for human use. The Celution® System, as an example, washes harvested lipoaspirate in a closed, presterilized, single-use consumable set to remove unnecessary red blood cells, clots, lipids, and extracellular matrix. The remaining tissue is digested with a proprietary pharmaceutical grade enzyme solution, Celase®. During digestion, nucleated cells are released from the tissue matrix and the cells are then concentrated and washed to separate the ADRCs from any lipid-laden adipocytes and the residual Celase® enzyme. In the end, a concentrated and ready-to-use ADRCs output is aspirated in a syringe for use in clinical applications. All reagents (isolation enzyme and buffer) used during the ADRCs isolation processing are medical grade, all procedures are well controlled in a closed system, and the final output is toxin free and pathogen free and contains a residual enzyme level that has been validated to have a high safety margin based upon common ISO biocompatibility standards.
ADRCs not only contain the same cellular composition as SVF [13] but also meet all safety and regulatory requirements for clinical use through standardized processing within a closed system. The process takes approximately 1 h and yields a highly viable output, providing physicians with the opportunity to deliver real-time bedside cell therapy to their patients. This safety and performance profile has enabled the Celution® System to receive regulatory approval in Europe as well as other regions around the globe. Currently, CE Mark indications include treatment for surgical and congenital soft-tissue defects, fistula, radiation wounds, and other ischemic tissue injuries.
The Celution® System is the best characterized [13] by the large collection of published clinical experience [61–73] in a broad variety of clinical indications from plastic and reconstructive surgery to general surgery and cardiology (Table 14.1). In the plastic and reconstructive field, an increasing number of physicians have started to combine ADRCs with fat tissue before grafting back to patients (Fig. 14.6). This approach provides physicians an option for better graft retention for soft-tissue filling applications, as well as in challenging wound healing indications.
Table 14.1
Published clinical studies and case reports by using adipose-derived stem and regenerative cells (ADRCs)
Indications | No. of patients treated | Study type | Administration and dose | Evaluation | References |
---|---|---|---|---|---|
Study of autologous fat enhanced w/ regenerative cells transplanted to reconstruct breast deformities after lumpectomy (RESTORE-2) | 71 | Prospective single arm trial | Deformities from breast conservation therapy (BCT) | Patient and physician satisfied with functional and cosmetic results | NCT00616135 [68] |
No local cancer recurrences during follow-up time period | |||||
Staged stem cell-enriched tissue injections for soft-tissue augmentation in hostile recipient areas | 29 | Case series | Local defects | Patients’ satisfaction with one single cell-enriched fat graft transplantation | [69] |
Treatment of “en coup de sabre” deformity with adipose-derived regenerative cell-enriched fat graft | 1 | Case report | Linear scleroderma | Patient and physician satisfied with functional and cosmetic results | [72] |
Autologous cell-enriched fat grafting for breast augmentation | 20 | Case series | Breast augmentation |