7 Technological Advances in Breast Surgery
Summary
Technological advances continue to impact the field of breast surgery. While implant technology is being refined, particularly with reference to cohesive gel development, some of the major changes taking place are those involving acellular dermal matrices and imaging techniques to assess skin perfusion. The purpose of this chapter is to review some of these changes together with the basic science behind them, which may impact surgical outcomes in the longer term.
Key Teaching Points
Acellular dermal matrix (ADM) can be helpful in controlling implant pocket shape.
ADM may reduce capsular contracture.
Preparation affects the biological performance of different ADMs.
Skin perfusion assessment is critical for immediate implant-based reconstruction.
Skin perfusion assessments may be helpful in determining flap viability during reconstruction.
Observations
This book is intended as a comprehensive yet practical resource for breast surgeons, providing data and algorithms to guide clinical practice. It also includes useful tips and innovative techniques to improve the quality and predictability of the procedures we perform. This chapter plays an important role in the book, because it focuses on an eclectic collection of techniques and products that may prove beneficial in solving some of the more intransigent problems that we face in breast surgery. These are advances that I have incorporated in my practice in recent years. Although I have no financial interest in these products, they have played an invaluable role in optimizing my results and clinical outcomes and in improving patient safety. As the use of these technologies expands, it is important for us to examine the benefits and limitations of these surgical adjuncts and to determine the appropriate applications.
7.1 Acellular Dermal Matrices by John R. Harper
7.1.1 Background
The use of regenerative acellular dermal matrices (ADMs) as an adjunct to breast reconstruction has received increasing attention in the United States during the past 15 years. The impetus for using these materials arose in response to surgeons’ frustration with existing solutions for reducing the problems and complications associated with expander- and implant-based breast reconstruction, including the following:
Capsular contracture.
Lack of lower pole ptosis.
Palpable and visible rippling of the device beneath the skin.
Window-shade deformity of the released pectoralis major muscle.
Infection, either immediate or delayed, resulting in loss of the device and failure of the reconstruction.
Attempts at reducing these problems have included the following:
Increasing use of cohesive gel implants.
Total subpectoral coverage of the device.
Marionette sutures to prevent window shading of the pectoralis major muscle.
Antibiotic lavage of implant pockets and the use of a no-touch technique to minimize skin contact with the implant.
Although the use of cohesive gel implants had been a major advance in other countries, until recently, their use in the United States was limited to clinical trials only. This has changed with Food and Drug Administration (FDA) approval for cohesive anatomical implant usage in recent years. The wrinkling of implants beneath the skin continues to be a significant challenge, particularly in thin patients. Capsular contracture remained an unresolved issue.
Window shading persists as a clinical entity, and total subpectoral coverage of the implant or expander tends to constrain the lower pole of the reconstruction, leading to a lack of ptosis in the final result. ADMs have provided a useful adjunct for the surgical management of many of these complex problems, helping to prevent or reduce their incidence.
Acellular tissue matrices have shown to be a useful adjunct to the plastic surgeon in the reconstruction of the postmastectomy breast, as well as revision surgery when postreconstruction complications occur. Plastic surgeons are accustomed to using the patient’s own tissues for reconstruction, always weighing the clinical benefits against the associated morbidity of harvesting grafts or elevating and transposing free or pedicle flaps to the recipient site. These are highly technical and time-consuming operations, and some patients are not candidates for these complex maneuvers because of their body habitus or various comorbidities that would put them at high risk for postoperative complications.
The goal of regenerative technology, such as acellular matrices, in managing these reconstructive challenges in breast reconstruction is to provide a scaffold that enables the surgeon to achieve excellent clinical outcomes while minimizing the morbidity of elevating the patient’s own tissues to achieve full muscle coverage. This approach has been shown to reduce the risk of the postoperative complications associated with skin breakdown and device exposure in reconstructive patients with compromised healing and breast skin.
In the past 15 years, numerous biological materials have been introduced for use in a variety of reconstructive surgical procedures. The premise is that biologically derived materials should allow the surgeon to achieve a better, more natural clinical outcome than synthetic materials. Despite the many choices in biological materials available to plastic surgeons, published data are often limited on most of these materials, and some confusion exists regarding the differences among them. Surgeons must have at least a fundamental understanding of these materials and their mechanisms of action so they can make educated choices when developing a reconstructive strategy. Numerous allogeneic and xenogeneic tissue scaffolds have been introduced commercially, and some are described in the literature (▶Table 7.1).
Alpha-gal | |||
Name | Company | Source tissue | Removed |
DermaMatrix | MTF (Synthes) | Human dermis | N/A |
FlexHD | MTF (Ethicon) | Human dermis | N/A |
AlloDerm | LifeCell | Human dermis | N/A |
Strattice | LifeCell | Porcine dermis | Yes |
SurgiMend | TEI Biosciences | Fetal bovine dermis | No |
Veritas | Synovis | Bovine pericardium | No |
Abbreviation: N/A, not applicable. |
7.1.2 Regeneration versus Repair
It has been suggested that during the first two trimesters of fetal development, the mammalian fetus can replace damaged or missing connective tissue through a regenerative process that does not involve scar formation. However, beginning late in the gestation period and throughout life, humans are intrinsically incapable of replacing lost connective tissue or increasing the amount or thickness of connective tissue without passing through a scarring or repair process.
The foundation of the repair process is the fibrin scaffold of the blood clot. This scaffold organizes the process. Inflammatory cytokines deposited within the fibrin structure trigger the inflammatory cascade, which involves robust cell ingrowth, angiogenesis, and enzymatic degradation of the scaffold. After collagen deposition during the fibrosis phase, the collagen is remodeled into scar tissue. Because the existing connective tissues are constantly undergoing an intrinsic tissue regeneration process, presumably driven in part by mesenchymal stem cells, it is conceivable that the proper scaffold could harness this capacity, enabling the body to regenerate tissue on this scaffold that is similar in structure and physiology to autologous tissue and superior to scar.
Therefore, the goal of using regenerative tissue matrices in reconstructive surgery is to establish an environment that enables the patient to regenerate tissue, other than scar or a foreign body capsule, mimics the autologous tissue, and allows the surgeon to achieve an excellent outcome with durable aesthetics and function. One key consideration for the surgeon in choosing the appropriate biological material for breast surgery is the patient’s response to the material. The most desirable response is one of minimal inflammation, which is properly regulated and resolves without resorbing the material. A chronic inflammatory response may lead to loss of the desired clinical result and the potential to generate contractile capsule or scar tissue.
7.1.3 The Goals of Using Biological Matrices in Reconstructive Breast Surgery and Characteristics of Currently Available Matrices
Reconstruction of the breast after mastectomy and approaches to revising complications that develop following cosmetic breast augmentation have evolved significantly over the past 20 years. Reconstruction involving tissue expanders followed by placement of permanent implants was originally described using total muscle coverage to minimize device exposure in the event of skin or incision breakdown. However, in order to minimize the donor site morbidity associated with raising the serratus anterior muscle or rectus muscle medially to achieve total muscle coverage, a dual-plane approach was later described in which the device was covered partially by the pectoralis major muscle superiorly and only the breast skin in the inferior and lateral aspects (▶Fig. 7.1).

When a surgeon uses the dual-plane approach, releasing the pectoralis major muscle inferiorly can result in cephalad rotation of the muscle, or window shading. This may result in displacement of the device inferiorly and laterally as well as thinning of lower pole skin. To avoid these complications, Spear and colleagues described the use of marionette sutures to control the position of the pectoralis muscle early in the postoperative phase to prevent window shading. However, inferior and lateral device migration and thinning of lower pole skin continued to be potential complications.
In addition, surgeons performing reconstruction with expanders or implants have struggled to produce satisfactory long-term aesthetic results because of the complications associated with the body’s response to the implants as foreign bodies. Capsular contracture and delayed infection requiring device removal or replacement present the patient and surgeon with the associated challenges and risks of additional surgical intervention. Therapeutic irradiation in mastectomy patients has also been shown to exacerbate some of these complications, especially capsular contracture. With respect to implant-related complications arising after cosmetic breast augmentation, surgical revision of many of these conditions can often be achieved by changing the plane of placement from subglandular to submuscular; changing the size of the implant; and, with the availability of silicone devices, exchanging saline-filled for silicone implants. In this case, moving to a dual-plane placement still risks the development of the complications just described.
Breast reconstruction using local autologous tissue such as the free or pedicle transverse rectus abdominis musculocutaneous (TRAM) flap, deep inferior epigastric perforator (DIEP) flap, and latissimus dorsi flap have avoided most of the device-related complications. However, these tissue transfer procedures are technically challenging and time consuming, and many patients are either not candidates for such procedures because of body habitus or elect to avoid the extensive surgery and down time. Moreover, management of the abdominal donor site in the case of TRAM flaps creates additional challenges (see Chapter 16).
Recent advances in device-assisted reconstruction using biological matrices have facilitated total tissue coverage without the morbidity of muscle dissection, while controlling the submuscular pocket against device migration laterally, superiorly, or inferiorly. The use of biological matrices permits surgeons to create a modified, dual-plane approach to give patients excellent aesthetic outcomes with natural-appearing breast ptosis and stable, defined inframammary folds, while covering the implants with vascularized tissue in the inferolateral aspect to prevent implant exposure in the event of wound dehiscence. The key element to success using a biological matrix in the lower pole is to use material that does not elicit a negative reaction in the patient, such as abnormal inflammation or a foreign body response that may lead to subsequent device-associated complications, including capsular contracture or device exposure and extrusion.
7.1.4 The Extracellular Matrix Directs Cell Behavior and Maintains Structure
In the early literature, the extracellular material of mesenchymal tissues was referred to as ground substance, because its complexity was poorly understood. Primarily composed of fibrillar type I and type III collagens, the extracellular matrix (ECM) also contains less-abundant components, such as small leucine-rich proteoglycans, fibronectin, several laminin species, glycosaminoglycans, and minor nonfibrillar collagens that contribute to the function of the ECM. In addition to the reticular basket-weave ECM found in mesenchymal tissue, epithelial tissues and capillaries also contain a lamellar ECM known as the basement membrane, which supports the epithelial layers of various mucosal tissues as well as the endothelial lining of blood vessels. The composition of the basement membrane is unique, with type IV collagen, fibronectin, laminin, nidogen, and heparan sulfate proteoglycans as the major components.
Cells interact with the matrix through a family of heterodimeric, transmembrane receptors called integrins. These receptors essentially integrate the extracellular environment with the intracellular machinery that regulates cell behavior. The interaction of these receptors with specific ligands within the ECM allows cell behavior to be responsive to growth factors and other biochemical and biomechanical signals present in the local tissue environment. When engaged with the matrix, second-messenger signaling molecules trigger transcriptional changes that ultimately lead to a change in a cell’s behavior, such as migration, expression of certain proteins, or even programmed cell death. By extracting ECM from tissues so that it will properly support a true regenerative response in humans, the structural and biochemical integrity of these ligands should be preserved as much as possible so that cells are capable of recognition and respond to the scaffold as self.
In the mid-1990s off-the-shelf ECM preparations were first shown to be a useful tool in minimizing donor site morbidity in surgical procedures that had previously relied on autologous tissue for reconstruction. It was demonstrated that an acellular dermal graft prepared from human allograft skin could serve as a scaffold for fibroblast and vascular ingrowth and support dermal regeneration in a full-thickness burn wound, minimizing the need for thick autograft donor skin. Later, these scaffolds were used in a variety of medicine, wound care, complex abdominal wall reconstruction, and breast reconstruction.
7.1.5 Processing of Connective Tissues to Produce Biological Tissue Matrices
Several important factors should be considered when producing a biological matrix for surgical reconstruction. Although surgeons need not have a deep understanding of these technical aspects, it is essential that they appreciate them in a general sense so that the impact of these factors on a patient’s reaction to the scaffold can be fully understood. The surgeon’s choice of scaffold should be based on both scientific and clinical data regarding performance of the matrix. The impact of processing on the device or tissue performance will be addressed in the following paragraphs.
7.1.6 Cell Removal
Removing donor cells and most of the potentially immunogenic cellular components is necessary to provide a graft that is not rejected by the patient, since the transplantation antigens or antigens encoded by genes of the major histocompatibility complex are the human’s first line of defense against implanted allogeneic or xenogeneic tissues. Cell removal has been accomplished by many methods, often involving detergents that solubilize cell membranes and intracellular components. Nuclear contents such as DNA and RNA often remain after detergent treatment but have not usually been considered a safety issue. In the case of animal-derived tissues, nucleases are often used to digest the nucleic acids into fragments that are washed away. Decellularization can be damaging to the ECM if the detergents or solvents used denature the fibrillar collagen or extract key noncollagenous proteins and proteoglycans. For example, decorin is a small, leucine-rich proteoglycan that “decorates” the collagen fibrils and has been shown to have several functions associated with the role of the matrix, such as growth factor binding and regulation, and regulation of the sensitivity of collagen fibrils to matrix metalloproteinase digestion. Decorin is easily denatured or even extracted from the collagen fibril by harsh detergents or chaotropic agents. This change in matrix structure could lead to macrophage activation that would trigger inflammation and resorption.
7.1.7 Addressing Xenogeneic Antigens in Porcine and Bovine Derivatives
In the case of animal-derived tissues, key xenogeneic antigens should be addressed to minimize or eliminate the cross-species immunologic reaction that would again lead to inflammation and resorption of the scaffold. One of these key antigens is a specific carbohydrate linkage on the N-asparagine–linked carbohydrates known as the galactose-alpha-1,3-galactosyl or alpha-gal component. This antigen is found on most lower mammalian tissues as well as on some bacteria and viruses and, because Old World primates have sialic acid instead of the alpha-1,3-gal, humans develop a constitutive humoral immunity against the alpha-gal structure. It is estimated that as much as 1%of the circulating immunoglobulin in an adult human is directed against this structure. If this antigen is not addressed in an animal-derived scaffold, it is likely to stimulate resorptive inflammation of the scaffold.
There have been several approaches to minimizing or eliminating the human response to this antigen on animal-derived tissue scaffolds. Most commonly, tissue scaffolds are treated with a cross-linking agent to mask the antigen from antibody recognition. This introduction of superphysiologic levels of covalent bonds within the scaffold renders the material resistant to recognition and breakdown by dampening the alpha-gal recognition. Permacol (Covidien) and CollaMend (Bard) are two examples of cross-linked porcine dermal matrices currently used in ventral hernia repair; however, there are no reports of these cross-linked scaffolds being used in breast reconstruction.
Enzymatic removal of the carbohydrate using a specific alpha-galactosidase is another approach that has been explored. Stone and colleagues have used this approach successfully in minimizing the immune response to porcine bone-tendon-bone implants for anterior cruciate ligament replacement. This strategy has also been used on a porcine ADM (Strattice; LifeCell/Allergan) to minimize the human immune response to the alpha-gal structure. The approach presently being explored in the xenotransplantation of whole organs is to use tissues derived from double alpha-gal knockout pigs. These animals have been genetically modified to silence or “knock out” both alpha-1,3-galactosyltransferase genes, which are responsible for encoding the enzyme that makes the alpha-gal linkage. The presence of alpha-gal on a commercially available animal-derived surgical mesh has been reported and is believed to be partially responsible for its rapid degradation in the body.
7.1.8 Preservation of the Scaffold for Storage
Preservation of the biological matrix for sufficient shelf-life and storage in the operating room is critical for the integrity of the ECM. The two most important considerations in the preservation and storage are matrix stabilization/preservation and control of microbial growth. Historically, various forms of freeze-drying or lyophilization have been used to stabilize biological matrices (AlloDerm [LifeCell] and DermaMatrix [Synthes]). If care is not taken to preserve the scaffold during the freeze-drying process or any other desiccation process, irreversible damage of the matrix can result and elicit a negative response in the patient. Alcohol storage (FlexHD; Ethicon) has been recently introduced as a medium for preserving human ADM and controlling microbial growth; however, careful analyses of the biochemical and structural integrity of a matrix stored in these conditions are needed. Most recently, custom solutions have been developed that effectively block potentially damaging effects of storage in a”ready-to-use” state, allowing surgeons to avoid the lengthy rehydration process involved in freeze-dried dermal matrices. This version of ADM storage is used in AlloDerm RTU (LifeCell/Allergan) and DermaCell (Novadaq/LifeNet Health).
7.1.9 Sterilization
The benchmark for sterilization is to achieve a sterilization assurance level (SAL) of 10-6 as determined by the bioburden of the material following processing. Most animal-derived tissues available today are sterilized by a variety of conventional methods, including gamma or electron-beam radiation and ethylene oxide. Additional techniques involve exposure of the material to a variety of antibiotic solutions, which then need to be eluted from the tissue using saline washes before insertion into the host. Little attention has been paid to the potential damaging effects of radiation on the performance of biological materials used in reconstructive surgery. The dose of these treatments required to achieve an SAL of 10–6 is determined by the bioburden of the material at the end of the process. When ionizing radiation is administered at standard sterilizing-dose levels, highly reactive superoxide radical species are generated that may cause unintentional cross-linking of adjacent proteins and some carbohydrates, in addition to cleavage of collagen. Furthermore, the oxidative condition created by ethylene oxide sterilization may lead to oxidative damage and modification of proteins and carbohydrates, causing matrix damage that will activate macrophages and trigger a degrative inflammatory response by the patient. The key step to achieving terminal sterilization with minimal matrix damage is to minimize or reduce the bioburden throughout the process so that the terminal step is capable of achieving an SAL of 10–6 with minimal free radical or oxidative damage. Antibiotic exposure does not damage the collagen elastin framework or denature protein. Although antibiotic-impregnated products could be challenged as being not truly sterile, the SAL bioburden of 10–3 is achieved and meets FDA standard for implantation similar to their irradiated counterparts. In some cases, terminal sterilization to an SAL of 10–6 has been chosen to avoid the damaging effects of higher levels of radiation (AlloDerm RTU, LifeCell/Allergan). Like many surgical procedures, in breast surgery, bacteria may be introduced into the surgical field through a variety of sources that are unrelated to the ADM or implants being used, such as residual bacteria from the glands of breast tissue following the mastectomy, skin contamination, foot traffic in and out of the operating room, and multiple staff over the field. Considering that the published postoperative infection rate in breast surgery with or without the use of ADMs is 4 to 6% (40–60 per 1,000 cases), an SAL of 10–3 does not introduce a clinically significant risk of infection or an SAL of 10–6.
7.1.10 Distinct Mechanisms of Action of Biological Matrices Based on Methods of Processing
The performance or mechanism of action of these matrices is determined by the process used to isolate the biomaterial from the source tissue, and it is incumbent upon surgeons and operating room personnel to have a general appreciation of these mechanisms. Three distinct mechanisms have been proposed based on advanced animal studies and confirmed through anecdotal human biopsies: regeneration, resorption, and encapsulation (▶Table 7.2).
These mechanisms can be segregated into positive and negative responses to the matrix. A positive response would be characterized as a quiet incorporation of the matrix into the patient’s tissues, with minimal or no clinically relevant inflammation or foreign body response. A regenerative matrix is a scaffold that supports fibroblast and vascular ingrowth and eventually a transition from the initial scaffold architecture to a tissue structure appropriate to the site of implantation. For example, AlloDerm has been shown to support lymphangiogenesis in animals, and may have a future role in treating patients with lymphedema. No studies have been published to date that evaluate the effects of other human ADM on lymphangiogenesis.
Tissue transition is the physiologic remodeling of an ECM with a given architecture into a structure that is orthotopically appropriate to the site of implantation. The hypothesis is that some cells migrating into the matrix may have mesenchymal stem cell properties that are responsive to local biochemical and biomechanical signals, resulting in remodeling of the scaffold into the appropriate structure. This has been illustrated in at least two instances in the literature. In the first example, a porcine analog to AlloDerm was used to replace a dural defect in a porcine model of duraplasty. By the 6-month postoperative gross and histological evaluation, the resulting tissue had a striking similarity to native dura instead of the original dermal structure. In the second example, dermal scaffolds (AlloDerm and Strattice) were used as a fascial replacement in the abdominal wall of a primate. Six months later, these were examined histologically, and the structure of the tissue demonstrated a greater resemblance to that of fascia than to the original dermal architecture. This positive reaction to the scaffold and subsequent maturation to an orthotopically appropriate tissue best exemplifies the regenerative mechanism.
If the ECM is damaged during processing, the graft can trigger a negative reaction, such as macrophage activation and uncontrolled inflammation, leading to breakdown and resorption of the scaffold. This may cause scar tissue or a classic foreign body response, resulting in encapsulation and extrusion. Several animal studies in a variety of species have attempted to examine the in vivo performance of biological matrices. However, the immunology involved in these animal models is sometimes ignored, and the data may not accurately predict a patient’s response to the material.
7.1.11 Animal and Clinical Studies That Address the Performance of Biological Matrices
Significant limitations are inherent in each animal model based on the scaffold being tested. Several rodent, rabbit, and porcine studies have been published describing the in vivo performance of a variety of biological materials. When evaluating these animal studies, it should be borne in mind that although the long-term in vivo response can be studied in a number of lower mammalian species, it is difficult to extrapolate these long-term results to the response in humans. This difficulty arises because of the chronic inflammation induced by the matrix, beginning between 4 and 6 weeks postoperatively. An example of this phenomenon occurs in human acellular dermal matrix (HADM), AlloDerm, studied in the rabbit model. Eppley described the rapid fibroblast and vascular ingrowth into AlloDerm in rabbits, noting that by 4 weeks, there appeared to be a chronic inflammatory response that eventually resorbed the material. Roth and colleagues have compared the early response to AlloDerm and FlexHD in a rabbit model. They reported that AlloDerm supported the ingrowth of immune cells in the rabbit to a greater degree than FlexHD. This is consistent with the rabbit data reported by Eppley; however, they did not offer an explanation for the lower level of this response in FlexHD specimens. Subsequently, rabbit and guinea pig models have been used to study only early events, such as fibroblast ingrowth, vascularization, and peritonealization that occur within the 4-week time frame. This absorption phenomenon does not occur in primates or human subjects.
The most appropriate animal system for attempting to model the response of humans to a biological matrix is a species that has high genetic and immunologic homology to humans. Recently, Sandor and colleagues have developed and characterized a model of abdominal wall reconstruction in the African green monkey (Chlorocebus sabaeus) for testing the immunologic and, in some cases, the functional response to biological matrices. Subsequently, Xu and colleagues compared several commercially available biological matrices for their in vivo response, and the data have served as the basis for proposing the mechanisms of action described previously. More recently, Connor and colleagues have used this model to evaluate the biological response to a non–cross-linked porcine ADM similar to Strattice in which the alpha-gal structure has been removed. The immune response of the primate to the porcine matrix is similar to that seen in humans in that the material was not inflammatory.
Judging from the cellular response to AlloDerm, compared with that of primate ADM produced by the same process, these animals respond to human matrix as though it is their own after 6 months, even though it is a xenograft. To date, this model appears to be the most appropriate system to characterize the long-term in vivo response to biological materials. It appears from these studies that if a matrix is well preserved in biochemical and structural integrity, a regenerative response is supported. Alternatively, scaffolds that exhibit varying degrees of damage or alteration as a result of the process used in production induce chronic inflammation or foreign body response, leading to scar formation or encapsulation. In order for surgeons to use both the scientific and clinical literature on the use of ADMs in breast reconstruction for making educated choices about which scaffold to use in their reconstructive approaches, they must gain a general appreciation of the concept of distinct mechanisms of action for biological materials.
Further work in the nonhuman primate experimental model has shown results with DermaCell, AlloDerm Freeze-Dried, AlloDerm RTU, and FlexHD Pliable. A detailed immunologic evaluation of the response of these animals the ADMs tested showed subtle, but significant differences in the levels of inflammation triggered by the materials. Since these materials are all produced from human allograft skin, these differences can only be explained by differences in the processing methods used, such as cleaning, sterilization, and storage conditions. While all of these materials are being used in implant-based breast reconstruction, there is little in the way of long-term data on longevity of the clinical outcome or histological consequence of the patient’s reaction. At the time of this edition, the data from primate studies and numerous clinical reports have demonstrated histological evidence of longterm persistence of AlloDerm Freeze-Dried and AlloDerm RTU in the lower pole of the human breast. Other materials are being investigated; however, long-term histological data are not yet available.
The long-term persistence of AlloDerm-derived tissue is shown at 2.5 and 5 years in the lower medial pole of the breast (▶Fig. 7.2). The distinct difference between this regenerated tissue and that of native breast capsule from the upper pole of the same breast is clearly seen. The matrix–implant interface is shown of a hematoxylin and eosin stain of AlloDerm placed in the inferomedial pole of the breast to revise implant position. In a Verhoeff–Van Gieson stain for elastin, the black bands indicate elastin from the matrix tissue placed 2.5 years before this biopsy. The elastin-negative tissue is scar tissue between the matrix and the skin of the breast. The high elastin content of the AlloDerm capsule may help to explain an emerging trend that suggests that these matrices may reduce capsular contracture around expanders and implants following immediate breast reconstruction.

Compared with the same stains of native breast capsule from the superior pole of the same breast, the distinction between regenerated subdermal tissue and capsule can be appreciated (▶Fig. 7.3a,b). One distinguishing feature of breast capsule described in the literature is synovia-like metaplasia at the tissue–implant interface. A cellular response to the implant is not generally seen at the interface of AlloDerm tissue and implant. Also, similar to most scars, breast capsule consists of collagen and does not contain elastin (right). One of the longest-term histological assessment of the biological integration and persistence of regenerated tissue on an AlloDerm RTU template was from a patient undergoing revision surgery unrelated to the original reconstruction by one of us (G.E.J., 5 years following the index reconstruction) exhibited normal dermal cellularity and vascularity, as well as remnants of dermal elastin (▶Fig. 7.3c,d).

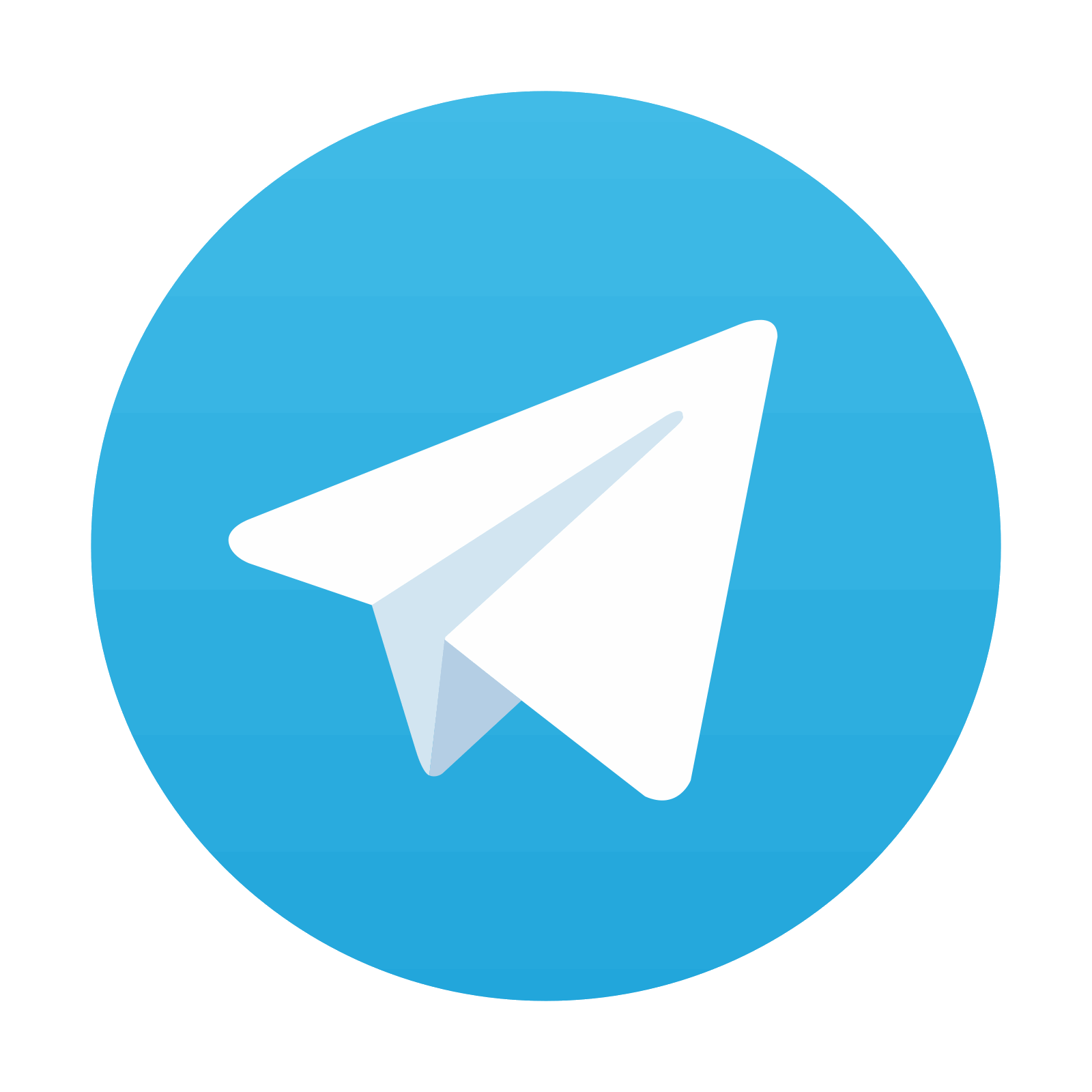
Stay updated, free articles. Join our Telegram channel
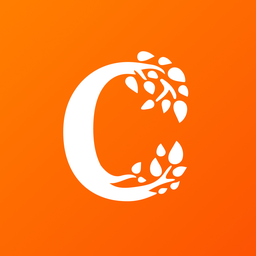
Full access? Get Clinical Tree
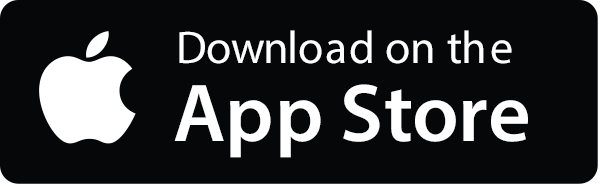
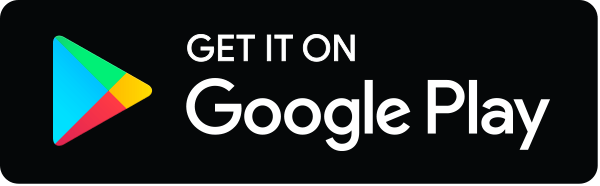
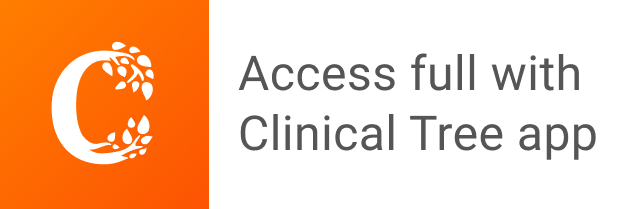