17 Tissue Expansion Abstract Reconstruction of soft tissue defects may be achieved by different methods. Skin grafts and flaps, either local, regional, or free, are all valid alternatives, depending on the case. However, they all produce varying degrees of morbidity on the donor area, may lead to a poor functional or cosmetic result in the case of skin grafts, and may be technically demanding. Also, in some cases, even these techniques may not provide enough healthy tissue for coverage. Tissue expansion is based on the use of a silicone device that is placed under the skin and gradually inflated with saline, thus causing stretching of the overlying tissue (skin, subcutaneous fat, fascia, muscle). Such forces favor cell proliferation and angiogenesis as well as the recruitment of neighboring skin so that at the end of the expansion process newly generated, well-vascularized tissue is available for reconstruction. Tissue expansion presents a spectrum of treatment options, including expansion of a skin graft donor site, expansion of neighboring skin to be used as a local flap, and expansion of distant skin to be used as a free flap. Regarding its clinical applications, tissue expansion may be used to treat soft tissue defects secondary to trauma, burns, oncologic resection, and congenital conditions, with postmastectomy breast reconstruction being one the most frequent indications. This chapter describes the main aspects of tissue expansion with regard to changes in tissues, surgical technique, potential complications, and clinical applications. Keywords: biological creep, implant, infection, stress relaxation, tissue expansion The capacity of tissues to stretch and expand gradually over time has been observed in physiologic and pathological circumstances, as well as being part of cultural ornaments. Some examples of physiologic changes of the skin and soft tissues may be seen during pregnancy, massive weight loss, and cranial development during the first years of life. Skin and soft tissues may also experience stretching and expansion as a consequence of pathological growth from underlying tumors, as may happen with soft tissue sarcomas and benign tumors from the skin or subcutaneous tissue. Classical examples of tissue expansion as cultural ornaments are seen in the Chad tribes, where people undergo lip stretching, or the Padaung tribe from Burma, famous for their elongated necks from the use of metallic rings. The history of tissue expansion did not begin with skin or soft tissue, but with the expansion of bones. In 1905 Codevilla and then Magnuson described bone lengthening using traction devices. In 1921, Putti, in Italy, demonstrated that sustained traction for a period of several months could lead to bone lengthening and also stretching of surrounding noble structures, such as nerves and blood vessels, preceding the method later described by Ilizarov. In 1957 Neumann used a rubber ball placed underneath the skin to obtain skin for cutaneous coverage of a subtotal ear amputation. Nevertheless, his results did not have much impact at that time. Two decades later, in 1976 and 1978, Radovan reported the use of a subcutaneous tissue expander that could be inflated percutaneously with normal saline through a remote valve. In 1979 and 1982, Austad and Rose described the use of a device that employed the osmotic gradient of sodium chloride for its inflation, which avoided the need for repeated injections. Despite this advantage, the long periods of expansion and the risks related to exposure of surrounding tissues to hypertonic solutions in case of rupture, made Radovan’s the preferred method for tissue expansion, which has remained to our days. Since its description, tissue expansion (TE) has had a significant impact on reconstructive surgery. The main advantages of TE include its capacity to provide additional tissue, with excellent blood supply due to increased blood flow, and to provide like-quality tissue in terms of contour, texture, and color match. Its chief uses include soft tissue coverage of traumatic and postoncologic defects as well as burn reconstruction, among others. Currently tissue expansion also has a major role in modern breast reconstruction and even microsurgery, where it has been applied for preexpansion of free flaps. Remember Radovan (1976) first reported on the use of silicone subcutaneous skin expanders. To date this is the most frequently used method of tissue expansion. The skin has viscoelastic properties, which determine that, in response to a stretching force, both the elastic and viscous components are affected. Collagen types I and III are produced by fibroblasts and represent the predominant proteins found in the dermis. In their natural state they are arranged as a triple helix, but under shear stress these fibers stretch to take a parallel disposition, resuming their original helical configuration once strain subsides. The biomechanical properties of the skin change with increasing age or under repeated stress. These properties determine the skin’s response to tissue expansion and are measured considering shear stress, elasticity, creep, and stress relaxation. Shear stress can be defined as the product of force and cross-sectional area, whereas strain (elongation) corresponds to the change in length in comparison with the original dimension. The relationship between stress and length can be represented as a stress–strain curve ( During the initial phases of strain the material becomes deformed in direct relation to stress until it reaches a point known as Young’s modulus or elastic modulus (E). Materials with higher values of E are more rigid than those with lower values. The equation that represents this relation is known as Hooke’s Law (σ = Ee) where σ is defined as stress and e as strain. Point A represents the elastic limit, so if a material is stretched beyond this point it will not be able to recover its original shape once stress is relieved, thus producing permanent deformity. As stress is reduced the elastic portion recovers, following a line that is parallel to the original curve and leaving a permanent deformity ( Remember Dermis collagen types I and III consist of a triple helix structure. During expansion these fibers adopt an increasingly parallel alignment. During tissue expansion the surgeon tries to optimize stress in order to produce the maximum stretching that the skin can tolerate. Accordingly the region under the curve between B and C must be considered, without exceeding the tensile strength of the skin, which would lead to its rupture, represented by area C, D of the curve. Skin can be stretched because of its inherent elasticity and as a result of biological and mechanical creep. Mechanical creep occurs when skin is stretched at a constant force, leading to extrusion of fluids from the interstitium of the collagen chain and subsequent skin stretching beyond its inherent elasticity. Apparently blood supply is not compromised during this process. A consequence of this phenomenon is stress relaxation, produced by the fact that if skin is stretched at a constant length the force required to maintain this state gradually reduces. On the other hand, biological creep is the slow and gradually expansion seen during pregnancy, weight increase, or tissue expansion, in which continuous stretching and tissue thinning stimulate production of new epithelium, collagen, elastic fibers, and neovasculogenesis as a means to reduce stress (stress relaxation). Remember Mechanical creep phenomenon occurs when tissue is acutely stretched beyond its inherent elasticity, whereas biological creep occurs during slow expansion (e.g., pregnancy, weight gain), where the stretching induces tissue regeneration. Upon expansion, different tissues (e.g., skin, muscle, bone, and the vascular network) experience a number of changes, which are summarized as follows: • Epidermal thickening and dermal thinning. • Formation of a capsule around the expander. • Muscle thinning and reduction in mass. • Bone thinning and new bone formation peripherally. • Mechanical strain triggering DNA synthesis and cellular proliferation. • Increased vascularity. The epidermis becomes thicker during tissue expansion. Initially this occurs as a consequence of tissue edema, which lasts for approximately 4 weeks; nevertheless, even after edema subsides, the epidermis remains thickened for several months. Conversely, the dermis becomes thinner, and changes in the dermal thickness last for at least 36 weeks after expansion has ceased. Total skin area increases from recruitment of adjacent skin and an increase in the mitotic rate. Hair follicles and appendages may become compressed but do not degenerate, and there is an increase in melanocytic activity, which reverts once the expander is removed. Following expander placement, a dense fibrous capsule is formed around the device, which shows increased vascularity and reduced cellularity as expansion progresses. Muscle always atrophies regardless of whether the tissue expander is placed above or below. Animal studies have shown that expansion induces growth of muscle cells and an increase in the total number of sarcomeres for each fiber of striated muscle. Once the expander is removed, muscle recovers its normal architecture, blood supply, and function. Bone density is not compromised; however, a decrease in its thickness is seen as a consequence of osteoplastic resorption, whereas peripheral bone may become thicker due to periosteal reaction. Bone density is not compromised. Cranial vault hollowing is frequent in children under 1 year of age, but it tends to recover after expansion has ceased. Long bones begin remodeling during the first 5 days after the expander is removed, reaching normalcy after approximately 2 months. Applying a mechanical stress on living cells affects a series of cellular structures and signaling pathways that lead to cell proliferation, growth, and tissue regeneration, which explains the formation of new tissue during the process of expansion. Mechanical deformation triggers a series of cellular responses involving the cytoskeleton, extracellular matrix, enzymatic activity, second messengers, and ionic channels. The cytoskeleton plays a major role in transforming extracellular forces into intracellular events, which are also transmitted to neighbor cells. Several growth factors essential for normal cell growth also appear to be involved in cell proliferation induced by stretching. These include epidermal growth factor (EGF), basic fibroblast growth factor (bFGF), transforming growth factor (TGF) family, platelet-derived growth factors (PDGFs), and angiotensin II. Activation of EGF receptors (EGFRs) generates an ionic influx that induces membrane ruffling, a phenomenon commonly seen previous to cellular division. TGF increases fibroblast growth and stimulates extracellular matrix production. In the absence of exogenous proteins, mechanical stretching by itself induces upregulation of growth factors, such as PDGF and angiotensin II, suggesting that tissue elongation may have an autocrine function too. The extracellular matrix is also essential for cell proliferation. Under mechanical forces there is an increase in DNA and collagen synthesis. In addition, the mechanical stress deforms the matrix, altering the configuration of adhesion proteins (e.g., integrins), which act as mechanochemical transducers, transmitting this extracellular signal to the intracellular environment by producing changes in the cytoskeleton. Several cellular morphological changes also occur as a result of rearrangement of actin filaments induced by mechanical stress. Actin also plays a key role in cell to cell signaling. Other families of transmembrane proteins, such as protein G, are also relevant for mechanotransduction in that their strain-induced conformational changes are supposed to initiate second-messengers signaling cascades and cellular growth. Ionic channels sensitive to mechanical stimulus favor the passage of several cations as Na+, K+, and Ca++ in response to strain, which promotes a diversity of secondary chemical reactions that determine cellular responses, including depolarization, cellular contraction, and generation of myogenic tone. Protein kinases, especially protein kinase C, are strong transducers of cellular signaling also favoring membrane ruffling. Some second-messenger systems, such as cyclic adenosine monophosphate (cAMP) take part in cell growth, differentiation, and protein synthesis. During mechanical stress the rate of protein synthesis is inverse to the concentration of cAMP, suggesting that this substance is consumed during such synthesis. In all, mechanical stress induces several cellular signaling pathways that lead to the expression of genes and proteins regulating cell proliferation ( Remember Mechanotransduction is the phenomenon by which cells convert mechanical stimuli into electrochemical signals; it plays a major role in the generation of new tissue during expansion. Vascularization is significantly enhanced by tissue expansion and is determined by an increase in the number and size of blood vessels. Angiogenesis, which is the generation of new blood vessels from preexisting ones, involves the microcirculation and occurs in response to tissue ischemia. Vascular endothelial growth factor (VEGF) is a known family of angiogenic factors and has been found to be elevated in expanded tissue following cyclic dermal stretching. From a clinical standpoint tissue expansion behaves similarly to flap delay because flaps raised on expanded tissue exhibit increased vascularity and higher survival rates and are usually of greater dimensions. Animal studies have shown a survival increase of 117% expanded versus nonexpanded flaps. However, it should be stated that fast expansion during the first 48 hours may compromise flap survival, and the rate of expansion should be guided by patient discomfort. There is a wide variety of tissue expanders, and their choice depends on the needs of each particular case. Originally, Radovan’s expander consisted of one silicone prosthesis with two valves connected to the main reservoir by silicone tubes, where one valve was employed for fluid injection and the other for fluid removal. There are now tissue expanders with integrated valves and remote valves, as well as self-inflating expanders, each with its own advantages and disadvantages. The basic design includes an empty reservoir made of silicone elastomer that is filled with fluid through an integrated or remote valve. Fig. 17.2 Effects of tissue expansion on surrounding tissues. Stretching of surrounding tissues induces growth factors, which mediate cell proliferation and increased angiogenesis. Factors such as transforming growth factor-β influence extracellular matrix production, whereas membranebound protein kinases, focal adhesion points, and cytoskeleton influence intracellular signaling cascades. EGF, epidermal growth factor; PDGF, platelet-derived growth factor; TGF, transforming growth factor. (Adapted from Takei T, Mills I, Arai K, Sumpio BE. Molecular basis for tissue expansion: clinical implications for the surgeon. Plast Reconstr Surg 1998;102:247–258. With permission from Wolters Kluwer Health, Inc.) Fig. 17.3 Schematic of possible signal transduction pathways induced by mechanical strain. A wide variety of strain-induced signals triggers sequential activation of transduction pathways and transmits signals through appropriate membrane receptor or ion channels. Terminal enzymes that are activated by these intracellular cascades transduce these signals into the nucleus. cAMP, cyclic adenosine monophosphate; EGF, epidermal growth factor; TGF-α and -β, transforming growth factor-α and -β; PDGF, platelet-derived growth factor; CTGF, connective tissue growth factor; IGF, insulin-like growth factor; PLC, phospholipase C; IP3, inositol-phosphate 3; PKA, protein kinase A; PKC, protein kinase C; DAG, diacylglycerol; MAPK, mitogen-activated kinases; MEKK, MEK kinase; MEK, MAPK kinase; JNK, c-jun amino terminal kinase. (Adapted from Takei T, Mills I, Arai K, Sumpio BE. Molecular basis for tissue expansion: Clinical implications for the surgeon. Plast Reconstr Surg 1998;102:247–258. With permission from Wolters Kluwer Health, Inc.) Expanders with integrated valves minimize the risk associated with the remote injection port and connection tubing, but in turn have a risk of puncture of the reservoir during injection when the integrated port is not adequately located. However, this has been simplified by incorporating metallic ports that can be readily identified by ultrasound or magnetic devices, the latter being very common in breast reconstruction. In 1982 Austad and Rose created a self-inflating device consisting of a silicone balloon filled with hypertonic sodium chloride (NaCl), creating an osmotic gradient that would allow its filling without the disadvantages associated with injection ports. These devices are now infrequently used because they require long periods of inflation between 8 and 14 weeks and have high rates of early rupture and tissue necrosis. K. Günter Wiese and then Shaheel Chummun developed expanders using an osmotically active hydrogel with the capacity to expand more than 10 times its original size. They consist of a polymeric matrix and an aqueous component, which absorbs fluid from the extracellular space causing edema of the hydrogel. They have the advantage of requiring fewer visits to the office, reduced pain, and faster expansion. However, their main downside is that expansion cannot be stopped in case of complications or doubts about tissue vitality. Expanders come in many shapes and sizes, which are determined by the characteristics of the defect and its location. Round expanders are frequently used for breast reconstruction, and crescent or rectangular expanders for the scalp or lower limbs. In addition, in some cases expanders may be custom made specifically for one patient/defect. There are also textured expanders as a means to reduce shifting and capsular contracture ( The main factors to consider during the preoperative planning of tissue expansion include the type, shape, and size of the expander, as well as the expected volume of expansion. Almost any kind of tissue is susceptible to expansion, but those with underlying bone tissue will have better results due to the transmission of the tensile strength of the expansion, preferably to the more compliant overlying skin, and not to the rigid bone. The presence of active infection, heavy smoking, and a previous history of radiotherapy are contraindications to tissue expansion given the unacceptable risk of complications. In addition, children under 7 years of age, expanders in the extremities, and integrated valve expanders in children have been identified as risk factors for complications; hence close surveillance during the expansion process is recommended in these cases. The use of expansion in trauma cases is advocated as a delayed rather than an immediate method of reconstruction. Careful preoperative planning is key for a successful tissue expansion, analyzing the location and size of the defect to be restored as well as the conditions of the surrounding tissues. For any given defect, it is generally better to use two medium-sized expanders rather than a single large one, as more tissue can be recruited using two devices. The base diameter of the expander should be approximately 2–2.5 times the diameter of the defect to be resurfaced. Additionally, a good estimate of the amount of tissue that can be advanced at the end of the expansion process may be calculated by subtracting the length of the base to the length of the circumference of the expander ( Surgical incisions should preferably be made at the margin between the defect and healthy skin to be expanded and, in the face, with attention to the aesthetic units and subunits. Closure should be performed without tension and in a layered fashion, including as many layers as possible (e.g., muscle, fascia, subcutaneous tissue) to prevent extrusion. Tension at the suture line may be reduced by placing the incisions radial or perpendicular to the long axis of the expander, though this is not always feasible. Careful hemostasis and the use of drains when necessary are paramount to reduce the risk of hematoma and seroma. Intraoperative expansion also serves as an aid to collapse small blood vessels. Selection of the type of valve (remote or built-in) depends on the surgeon’s preferences and each particular case. Remote valves should be placed far from the expander to avoid superposition of both during the expansion process. Also, they should ideally be superficial enough to allow easy identification, over a hard non-weight-bearing surface and fixed with sutures to avoid migration and kinking. Of note, bacterial colonization of the capsule has been reported in up to 82% of expanders with remote valves. Following insertion, intraoperative inflation up to 10–15% of the total volume may be performed, which helps to fill dead space and reduce risk of hematoma/seroma. Two to 3 weeks after placement and provided that all wounds are healed, expansion can begin on a weekly basis (or twice weekly in children with less volume infiltrated on each session), injecting approximately 10–15% of the total volume on each session. During expansion, it is important to pay attention to the development of pain and skin blanching because these are hallmarks of ischemia. Overexpansion up to 25% of the device’s volume is usually possible and may be performed to obtain enough skin coverage. Once the desired volume is reached, the second stage is planned, during which the expander is removed and the flap advanced into the defect. At this stage, scoring of the capsule can be done to allow improved mobilization of the flap. Remember • Careful planning is key for a successful tissue expansion. • Incisions must be placed close to the defect, not the expander, and as perpendicular as possible to the long axis of the expander. • During expansion, infiltration should be stopped before pain appears. • Over expansion is possible in case extra skin is needed. • Scoring of the capsule allows improved advancement of the flap.
17.1 Introduction
17.2 Basic Science
Fig. 17.1).
Fig. 17.1 dotted line). The limit of an elastic behavior is known as point B, or yield strength. If stress is applied between the limits of the plastic region, an increase in stress will produce a nonlinear increase in the stretching of the material (
Fig. 17.1 points B, C). In case stress is sustained it will reach a point (
Fig. 17.1 point C) in which the tensile strength of the material is exceeded, followed by accelerated stretching and rupture.
17.2.1 Tissue Changes during Expansion
17.2.2 Cellular Changes and the Effect of Tissue Expansion on Blood Supply
Fig. 17.2 and
Fig. 17.3).
17.3 Types of Expanders
Fig. 17.4).
17.4 Preoperative Planning and Surgical Technique
Fig. 17.5). In terms of shape, certain expanders are more suitable for specific areas, such as round or anatomically shaped devices used for breast reconstruction, and rectangular and crescent-shaped devices used in the scalp and extremities. Routine antibiotic prophylaxis is recommended to minimize the risk of infection.
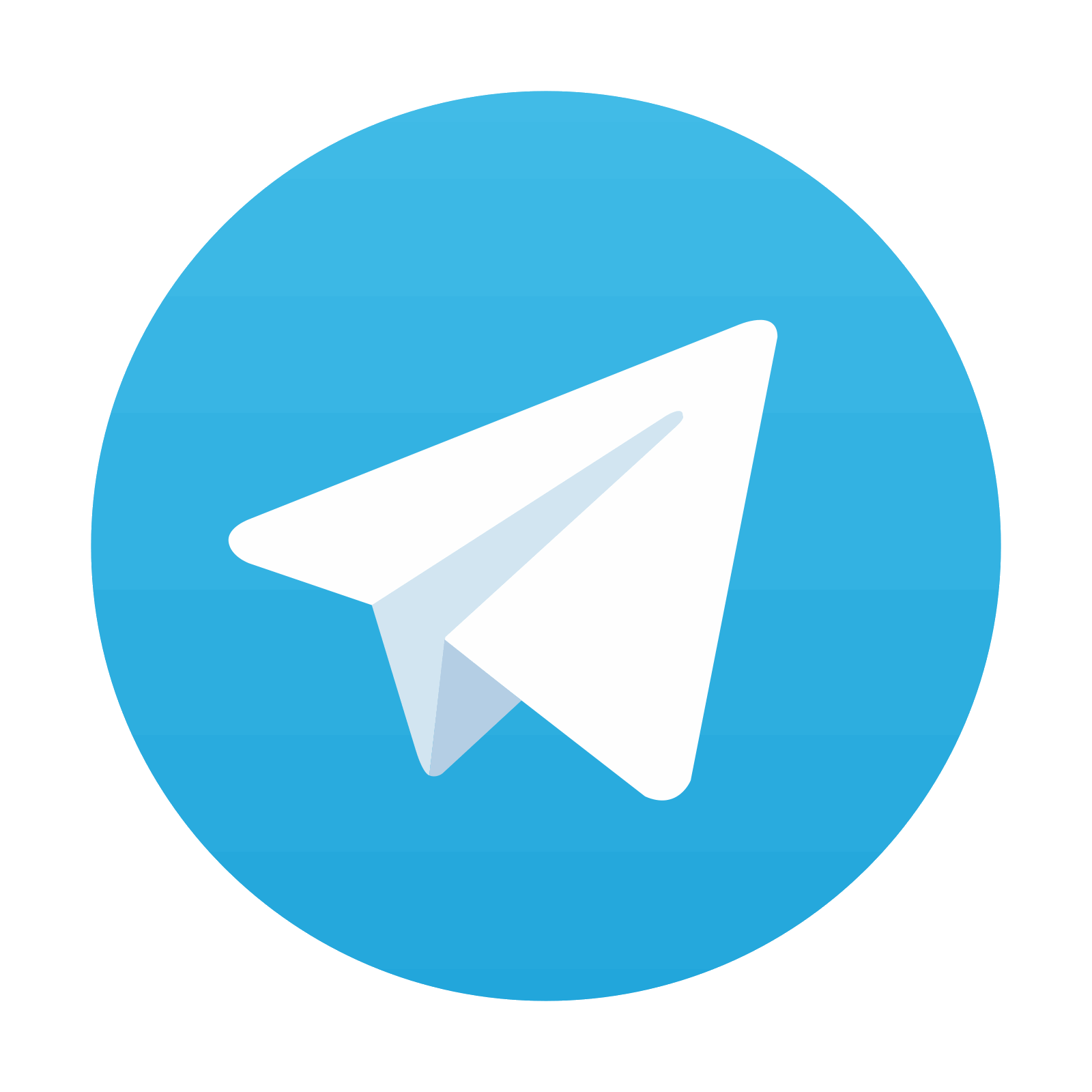
Stay updated, free articles. Join our Telegram channel
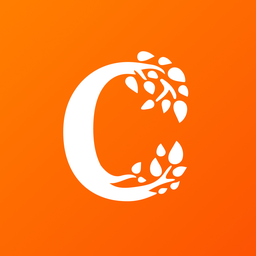
Full access? Get Clinical Tree
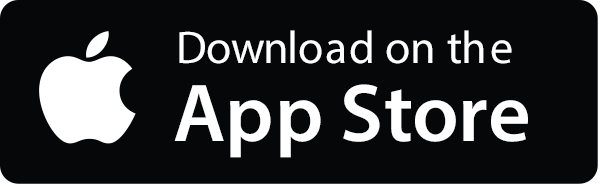
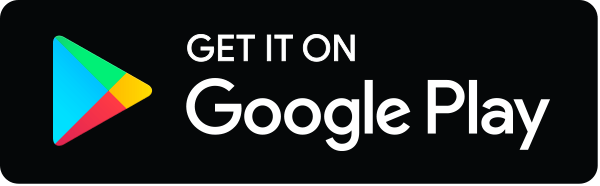