Introduction
The reconstructive options for today’s plastic surgeons are unprecedented in history. Despite this reality, the ideal reconstruction for many patients and defect types does not yet exist. The traditional paradigm of plastic surgery has centered around re-purposing existing tissues through grafting, locoregional or free flap transfer to replace “like with like” in keeping with the principles of Sir Harold Gillies. Re-purposing existing tissues is an elegant solution that serves us well and may be augmented through the use of prefabrication and prelamination techniques, but the ideal reconstruction would replace “same with same” and thereby avoid donor site morbidity entirely. Recently, composite tissue allotransplantation (CTA) has demonstrated its ability to replace “same with same” through the use of tissue and organ donors for facial, limb, and other transplants. CTA is directly analogous to organ transplantation, a discipline that owes much of its origins to Dr Joseph Murray, a plastic surgeon and Nobel laureate, who pioneered live related organ donation over 60 years ago. Both organ transplantation and CTA patients carry a significant burden in terms of the sequelae of long-term immunosuppression including rejection, graft versus host disease, opportunistic infections, and increased incidence of certain cancers.
Tissue engineering (TE) offers a means to grow replacement tissues and organs, thereby replacing “same with same” to restore form and function to the premorbid state. Patients with defects from trauma, cancer, burns, and often complex congenital conditions could all benefit immensely from advances in tissue engineering through the provision of improved and less morbid options for their management. In addition, tissue engineering has obvious benefits for esthetic surgery due to its potential to effect changes in skin texture, contour, tissue volume, and general appearance. Plastic surgeons already have significant expertise in the surgical manipulation of human tissues and organs. Tissue engineering builds on this foundation to form part of the present and future of plastic surgery.
Tissue engineering (TE) has been variably defined. The National Science Foundation in 1987 developed the following definition : “Tissue Engineering is the application of the principles and methods of engineering and life sciences towards the fundamental understanding of structure-function relationships in normal and pathological mammalian tissues and the development of biologic substitutes that restore, maintain, or improve tissue function.” More simply, tissue engineering is a discipline that utilizes cells, matrices, growth factors, and biomaterials to generate new tissues and organs for clinical use including multiple organ systems and tissues ( Fig. 7.1 ). It is also used as a research tool to further our understanding of the interactions between these components in both health and disease states. Unfortunately, tissue engineering techniques are not yet able to replace organ transplants in transplantation surgery. However, the past decade has seen an exponential increase in the implementation of tissue engineering strategies in clinical practice. During this time, plastic surgeons have played a key role in the development and implementation of these techniques and various biomaterials in surgical practice.

Plastic surgeons may function as the primary drivers of advances in tissue engineering or they may partner with engineers and/or cell biologists to translate promising laboratory research from the bench to the bedside. Engagement of clinicians in research is vital to advancing the field of tissue engineering. It provides a means to address unmet clinical needs and it fosters the innovation that continues to expand our scope of practice in an age where our specialty is being undermined at the edges by other specialties. As tissue engineering becomes a more routine component of clinical practice, it is essential that every practicing plastic surgeon has a working knowledge of its principles. Here we outline tissue engineering as a discipline, describe its approaches, and highlight the emerging technologies that are likely to transform our specialty over the coming decades.
Experimental Tissue Engineering
Tissue Engineering as a Tool to Further Scientific Discovery
The common misconception about tissue engineering is that it is focussed solely on the production of new tissues and organs to replace lost or malfunctioning body parts. In practice, it is also used widely as a tool for scientific research. Tissue engineering techniques can recapitulate specific aspects of tissues and organs to permit the investigation of structure–function relationships and the testing of therapies such as new medicines. Both beneficial and maladaptive interactions between cell populations (including stem and progenitor cells) and their local environment can be studied across multiple different platforms (in vitro, ex vivo, and in vivo). There are manifold reasons that tissue engineering can be a useful research tool in plastic surgery. Conditions such as hypertrophic and keloid scarring do not occur in laboratory animals and therefore tissue engineering approaches are useful to approximate elements of these conditions and to develop and test novel therapies. Furthermore, many conditions that are treated in plastic and reconstructive surgery are not life-threatening and therefore it can be difficult to procure funding for expensive animal studies or human trials. Our management of human aging is difficult to replicate in experimental animals and therefore preclinical tissue and organ aging research requires suitable models to bridge the gap between basic research and human trials.
There are many tissue engineering techniques used experimentally to further plastic surgery including, three-dimensional (3D) cell culture/multicellular organotypic culture and tissue- and organ-on-a-chip technology where the local environment of multicellular constructs is tightly controlled. Worldwide, many laboratories use such techniques to examine conditions of relevance to our specialty, such as the effects of diabetes mellitus on wound healing, the survival of ischemic tissues, and Dupuytren’s contracture amongst others. An in vitro model of hypertrophic scarring was developed in our laboratory using tissue engineering principles. A collagen gel seeded with fibroblasts is placed under tension to replicate the conditions for human hypertrophic scar formation ( Fig. 7.2 ). This model has provided invaluable insights into the interaction between cells and their matrix in hypertrophic scarring and facilitated the subsequent development of a murine model. These models provide numerous opportunities for further study as they allow the use of technologies that are not readily used in humans, including gene editing, transgenic organisms, and viral transfection.

Applied Tissue Engineering – a Team-Based Discipline
Applied tissue engineering focuses on the translation of laboratory-based tissue engineering techniques into the clinical setting (“from bench to bedside”). Just as multidisciplinary teams are used for management of complex cancer cases, a team approach is often ideal for the development of new tissue engineering techniques in a clinically relevant manner. Plastic surgeons are well placed to partner with engineers and cell biologists to advise on such translational research and clinical trials based on the broad spectrum of our practice (both anatomically and in terms of subspecialty), skills in microvascular and peripheral nerve surgery, and our expertise in identifying unmet clinical needs. Without such partnership, much of fundamental cell science and biomaterials research does not translate appropriately into the clinical setting. Even highly publicized laboratory-based tissue engineering research can fail to translate to the clinical setting when the tissue engineered construct meets an intact whole organism, particularly when it has to interact with an intact immune system. Pioneering ear cartilage tissue engineering research by Langer and Vacanti excited the public and garnered both significant interest and funding for tissue engineering research but it did not directly translate in immune-competent animals, including humans ( Fig. 7.3 ). Such research outcomes emphasize the need for partnerships and team-based approaches in tissue engineering that leverage the expertise of the group to achieve clinically relevant outcomes. Commercialization of successful research also requires a different, yet complementary skillset, but luckily there are improving pathways for commercialization through public and private institutions including universities, hospitals, and incubators. The current expertise in all of these fields makes tissue engineering an exciting and collaborative endeavor that can reap real rewards for patients.

Tissue Engineering in Plastic Surgery
The long history and problem-solving nature of plastic surgery has resulted in the development of a number of techniques that can be considered as forms of tissue engineering. Autologous fat grafting is a possible example. It has been used for over a century to address contour defects or provide additional volume. Our early understanding of its mode of action was that the adipocytes were simply being transferred and engrafted at the recipient site. A more nuanced understanding of this technique involves multiple overlapping processes that also include cell ischemia with chemokine signalling, inflammation, stem cell transplantation (adipose-derived stem cells– ADSCs) and recruitment of local and systemic stem cells. From a tissue engineering perspective, it involves the transfer of stem and progenitor cells within their native matrix along with parenchymal cells. A number of mechanistic studies demonstrate that both engraftment and adipogenesis occur to varying degrees depending on both local and systemic factors. Yoshimura and colleagues proposed a more tissue-engineering-like approach through the addition of ADSCs to the lipoaspirate (cell-assisted lipotransfer) in an effort to improve engraftment and volume retention. Whether this is beneficial is still uncertain. The addition of platelet-rich plasma (PRP) to lipoaspirate demonstrates some benefits over lipoaspirate alone for volume retention long term. There is mounting evidence that autologous fat grafting may improve local vascularization and tissue quality in studies where it has been used underneath irradiated skin. , The exact mechanism for this effect is unknown but the data argue for a role for autologous fat grafting as a form of tissue engineering, despite ongoing debate as to whether de novo adipogenesis, simple engraftment or lipid cyst formation occurs in any given site or individual. Research to improve its reliability, long-term volume retention, and our understanding of its mechanism of action are ongoing.
Another clinical technique that uses tissue engineering principles involves the insertion of silicone rods (eponymously referred to as Hunter’s rods) for the generation of a neo-sheath for secondary flexor tendon reconstruction in hand surgery. Where a primary tendon repair has not been possible due to tendon shortening or contracture of the flexor tendon sheath, secondary tendon reconstruction with the use of a tendon graft becomes necessary. To achieve a functional reconstruction, it is necessary to reconstitute a glide plane for the tendon in the form of a neo-sheath. In this setting, a two-stage procedure is performed whereby a smooth, silicone rod with a roughly semicircular cross-section is inserted along the line of the required neo-sheath. Within weeks, encapsulation of the silicone occurs and once the silicone is removed, this forms a smooth tunnel for the tendon reconstruction to glide through during healing and active recovery.
There are now increasing numbers of commercially available tissue engineering products in plastic surgery. Some obvious examples include skin/dermal substitutes for burns and wound care, nerve conduits/wraps and acellular biomaterials for coverage of implants and hernia repairs. It is unsurprising that skin/dermal tissue engineering has one of the longest histories in plastic surgery due to the vital need for skin and dermal substitutes in acute and chronic burns patients and the relative ease of creating somewhat two-dimensional structures. Skin tissue engineering was one of the first published studies within the field. , Despite the commercial success of many of these products, there still does not exist an “off the shelf” substitute for a split-thickness skin graft, highlighting the ongoing need for translational tissue engineering research.
Irrespective of the type of technique, tissue engineering strategies share some common elements. They typically involve a cell source (either autologous or allogeneic) and a biomaterial of some sort (either exogenous or induced/endogenous) with the end result being new tissue or organ production. This may be achieved through the use of a mixture of cells and biomaterials and there remains significant overlap between tissue engineering, stem cell therapies, and the implantation of biomaterials.
From a regulatory perspective, tissue engineering strategies are stratified by whether they are cell-based or acellular and by whether they incorporate biological matrices and/or have synthetic components. Greater regulatory hurdles exist for cell-based applications and there are correspondingly fewer cell-based products available for clinical use. Similarly, the use of materials that already have regulatory approval (e.g., components already used in clinical practice such as suture material or collagen) have an easier pathway to regulatory approval and are therefore found in more currently available products. Despite these hurdles, cell-based constructs can demonstrate excellent outcomes relative to non-cell-based applications, particularly when the patient or their recipient site have comorbidities (e.g., diabetes mellitus) that limit local recruitment of cells and/or the suitability of the recipient site. In practice, plastic and reconstructive surgeons have a wide range of clinical experience with tissue engineering products and therefore are well placed to take an active role in the development and implementation of new tissue engineering strategies in clinical practice. A thorough grounding in the basic principles of tissue engineering significantly helps us as a specialty to perform this role.
Targeted Tissue Engineering Strategies
Early attempts to grow specific tissues relied upon a subcomponent approach to tissue production. This empiric approach put together the subcomponents of tissues or organs in the hope that they might self-assemble into a useful structure in vivo. Sadly, this was rarely the case, but many useful insights were gained by considering the important subcomponents of specific tissues and organs and how they interact. Developmental studies examining cell interactions during tissue and organogenesis have improved our understanding of how new organs and tissues form naturally but they are of limited benefit for the production of new tissues clinically as adult stem cells demonstrate lineage restriction and it is difficult to replicate the fetal milieu ex utero. Tissue engineering researchers instead looked to fields where new 3D tissues grow in adult life. Tumorigenesis is probably the best example of this and many insights into tissue engineering have been gained by examining the process of tumor formation.
Neovascularization in Tumorigenesis and Organogenesis
There are numerous parallels between the study of solid tumors and the discipline of tissue engineering, with the exception that the goal of each of these fields is different (inhibit cancer growth versus stimulation of new organ growth). Much of our understanding of postnatal tissue growth owes its genesis to oncology researchers. Judah Folkman, a surgeon, established the need for new blood vessel growth (angiogenesis) for cancers to grow in three dimensions. Each cancer cell must remain in close contact with the blood supply, and the cells and the vasculature must form a mutually supportive ecosystem.
New tissue growth requires new blood vessel formation (neovascularization). Two types of neovascularization have been identified. Vasculogenesis is the de novo formation of blood vessels from vessel progenitors. It is an in utero phenomenon, but it may make a small contribution to neovascularization in the postnatal setting under certain circumstances. Angiogenesis is predominantly a postnatal process where new blood vessels sprout from existing blood vessels under the influence of local factors (growth factors, chemokines, and physicochemical gradients) ( Fig. 7.4 ). Angiogenesis is the predominant process in adult animals for new blood vessel formation and is one of the major targets of cancer therapies and tissue engineering strategies.

The vasculature of tissues changes under the influence of stimulators or inhibitors of angiogenesis. This process starts with signaling that increases vascular plasticity, typically under the influence of factors such as angiopoietin-1. The vasculature may then increase or decrease in branch points, vessel length, and vessel diameter, depending on the relative balance between pro-and anti-angiogenic signals. While new blood vessel formation is important, its regulation is just as important. Tissue engineering uses pluripotent and multipotent stem cells that are capable of forming multiple different types of tissue. The degree of vascularization within their microenvironment may influence their differentiation during the formation of tissue engineering constructs. For example, bone formation occurs readily in well-vascularized settings whereas cartilage formation is promoted in less vascular microenvironments. For this reason, pro- and anti-angiogenic signals must be tightly controlled both spatially and temporally during tissue engineering. Angiogenesis also supports tumorigenesis. Therefore, the stimulation of angiogenesis for tissue-engineering may pose a risk for tumorigenesis. For example, the stimulation of angiogenesis by adipose-derived stem cells (ADSCs) is one putative mechanism behind some reports of increased tumor recurrence following autologous fat grafting, particularly with c-MET-expressing breast cancer cell lines in animal models. This has not been established in humans but such data remain a concern for tissue engineers in the long term.
At a stable tumor volume, tumor cells and endothelial cells send stimulatory signals to support each other, thereby maintaining total tumor volume. When tumor growth exceeds the ability of the vasculature to support it, focal tumor necrosis occurs. Normal tissues display co-regulation between the parenchyma and the vasculature with long-term maintenance of tissue or organ volume being dependent upon a stable vasculature. This is in contrast to wound healing responses where new blood vessels are formed to support tissue healing and scar formation but the new endothelial cells undergo apoptosis over months to years to return the site to a near pre-wounding vascular density and volume. For tissue engineering strategies to demonstrate their effectiveness, they have to be able to generate the required tissue and maintain that volume long term, rather than representing some form of aberrant wound healing response. The effectiveness of tissue engineering strategies must therefore be assessed over the long term and this is not often done effectively in experimental tissue engineering. Additional insights can be gained from such studies and can help direct studies in humans. Inflammation is a potent source of pro-angiogenic factors but when excessive it may promote scar formation instead of the target tissue. There are numerous cells in the local microenvironment that contribute to the regulation of inflammation and their regulation is an important consideration in tissue engineering. The control of local inflammation will be discussed later in the chapter when we consider the local microenvironment for tissue engineering.
Seed versus Soil Hypothesis
Another parallel between oncology and tissue engineering is the ‘seed versus soil’ hypothesis. This was originally described by Stephen Paget as a way to understand tumor metastases. In the present context it refers to whether the “seed” (stem/progenitor cells) or the “soil” (the local microenvironment) is the most important factor in the generation of new stable tissue constructs. The seed hypothesis focuses on the capacity of stem and progenitor cells to adapt to and alter their microenvironment to generate new vascularized tissue. The primacy of stem and progenitor cells in new tissue generation is well known but soil protagonists argue that the creation of a suitable microenvironment triggers the recruitment of endogenous stem and progenitor cells and provides the cues for them to replicate, differentiate and vascularize to generate a stable tissue construct. A composite view considers the needs of a stable stem cell niche and how this can be manipulated to facilitate the formation of new specific tissue in a controlled manner with reconstitution of a stable stem cell niche within the target tissue following completion of the necessary tissue growth. Where limitations to endogenous stem and progenitor cells may exist (patients with comorbidities including aging, diabetes mellitus, and irradiation), greater focus may be required on “seed” approaches that can compensate for deficits in stem/progenitor cell function and/or trafficking. Where normal stem and progenitor cell function is present, “soil” approaches can be very successful (e.g., young healthy animals). Both approaches rely upon the ability to temporarily bypass the normal control mechanisms that limit the formation of new tissues (and/or benign tumors). The control mechanisms that limit stem cell division and differentiation under normal physiological conditions are still incompletely understood. The risk of forming cancers remains a stigma for tissue engineering, despite little evidence to establish that tissue engineering techniques significantly increase the risk of cancer. As more is understood about these regulatory mechanisms, this uncertainty should decrease.
Stem and Progenitor Cells in Tissue Engineering
Stem cells are long-lived cells that are capable of a number of important functions, including the ability to form multiple different tissue types, encourage or participate in new blood vessel formation (neovascularization), immunomodulation and self-renewal. Progenitor cells are usually daughters of stem cells that are already committed to a given cell fate (not multipotent) but are capable of both self-renewal and reconstituting their given cell/tissue type. Endothelial progenitor cells (EPCs) are a common progenitor cell type used in tissue engineering because of their putative ability to develop into vasculature/endothelial cells. Combinations of stem cells and endothelial progenitor cells are often used to support the coevolution tissues with their supporting vasculature.
Broadly, stem cells are divided into embryonic stem cells (totipotent or pluripotent), postnatal stem cells (multipotent), and induced pluripotent stem cells (iPSCs). Embryonic stem cells are a valuable tool in the study of developmental processes and fundamental aspects of stem cell biology, but they have significant ethical issues as they need to be harvested from embryos, often secondary to the retrieval of the embryo from the uterus. This process is usually destructive of the embryo and therefore poses significant ethical problems and is not a readily available option for tissue engineering in conventional care settings. Postnatally, stem and progenitor cells are present in most tissues of the body and continue to have important roles in tissue homeostasis based on their support for cell turnover (with a more limited capacity to form multiple tissues), immunomodulation, vascularization, and self-renewal ( Fig. 7.5 ).
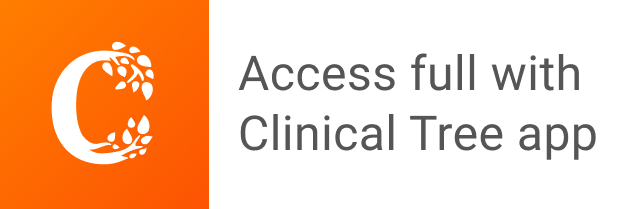