Key points
- •
The plastic surgical axiom of replacing ‘like with like’ should be the goal in the use of aesthetic fillers.
- •
Autologous fat grafting (AFG) best follows this axiom for many indications.
- •
Clinical success of AFG is limited principally by disparate techniques, resulting in variable graft quality, patient variability, and biological limitations of grafting.
- •
Combinations of fat graft and the addition of adipose-derived regenerative cells (ADRC) appear to enhance graft retention.
- •
Additionally, standardization, systemization and improved tissue handling appear to enhance the effect of ADRC and improve results.
Today’s aesthetic fillers: state of the art
A plethora of facial aesthetic filler options are available for dermatological and surgical use. Over 30 manufacturers of more than 90 filler products were identified in a recent consumer guide ( ). The composition of these fillers can be separated into two broad and overlapping categories, dermal fillers and soft tissue fillers. As shown in Table 9.1 , dermal and soft tissue fillers address different aesthetic needs and clinical indications.
Dermal fillers | Soft tissue fillers | |
---|---|---|
Aesthetic needs | Repair of age related small and medium sized wrinkles, volume restoration of lips and nasal/labial folds, burns | Repair deficits of subcutaneous adipose depots |
Etiology | Age, UV radiation damage | Local or systemic lipoatrophy, tissue lost from traumatic injury |
Current solutions | Hyaluronic acid, collagen, other organic polymer based injectables, allogeneic acellular grafts | Autologous fat graft |
Deficiency of current solutions | Filler retention time too short (requires multiple procedures to maintain; foreign body/immunologic response | Technically demanding and variable outcomes. Long-term retention/volume loss, cell necrosis, replacement of adipose with fibrotic matrix |
Dermal fillers are optimal to repair small to medium size wrinkles and defects resulting from age-related changes in dermal/subdermal musculature interactions within the skin. The majority on the market today are hyaluronic acid-based injectable gels. Collagen, organic polymers or minerals such as tri-calcium phosphate or hydroxyapatite comprise the foundations of the majority of other marketed fillers. To address both technical and patient oriented concerns, manufacturers of aesthetic fillers have tried various permutations of these components to address deficiencies of previously available products. Artificially synthesized biopolymers or human tissue derived biomolecules have been used as alternatives to animal-derived proteins. Manufacturers have addressed issues such as ease of use, retention in the application site, and foreign body response by altering filler particle size, bioresorption profiles and immunogenicity. Regardless of the dermal filler used, the dominant deficiency of the available products is retention time since the biocompatibility of the fillers also predisposes them to removal by the body’s immune and maintenance systems. Only one, Isolagen®, currently utilizes viable cells (autologous cultured fibroblasts) for wrinkle and fold repair which could theoretically overcome the body’s propensity toward resorbing foreign substances and remodeling extracellular matrix.
Soft tissue fillers target the loss of subcutaneous fat resulting from acute or chronic adipose tissue atrophy, surgical removal or injury. Historically, a number of synthetic fillers such as paraffin, rubber, latex and silicone have been used; however, both patients and physicians alike prefer natural ‘like for like’ solutions for treating dermal or soft tissue defects. Autologous fat grafting (AFG) has been used as such a solution in a wide variety of aesthetic and clinical indications to address issues associated with ‘artificial’ fillers. The use of AFG for aesthetic defect repair is not a new idea. The first reported use of AFG is documented in the medical literature of the late 19th century ( ). However, even with the advent of modern adipose tissue collection methodologies in the 1970s, the reported success of AFG for aesthetic facial repair has been mixed ( ). Table 9.2 describes both technical and biological factors that contribute to the mixed success of AFG. More comprehensive analyses of each factor’s role in the successful use of AFG are described in recent reviews by Coleman ( ) and .
Factors | |
---|---|
Pre-harvest |
|
Harvest |
|
Graft processing |
|
Transplantation or injection |
|
Both reviews identify methods of tissue collection and transplant technique as critical factors for AFG success. However, biological factors predominate when AFG is used for larger volume applications. Importantly, if graft volumes are greater than 3 mm 3 after the tissue is injected; internal regions of the graft are subject to periods of oxygen deprivation and nutrient deficiency due to limits in nutrient and oxygen diffusion until the onset of tissue revascularization. Untimely delays in new graft angiogenesis may likely result in cellular necrosis, induction of apoptosis, and secondarily, extensive edema, formation of lipolytic cysts, and ultimately tissue volume loss. Another important biological variable of AFG success is the amount and rate of tissue remodeling. Depending on graft preparation methods, adipose graft tissue has been observed to be replaced with fibrotic matrix over time in murine models of AFG ( ).
Thus, while there are many filler options available to today’s aesthetic surgeons there remains much room for improvement. The ideal filler would be easy to work with, biologically compatible, demonstrating long-term retention (greater than 1 year), and its use would not be limited to small volume applications. The discovery of adult stem cells and in particular, adipose derived adult stem cells (ADSCs) provides a biological solution to the important challenges associated with current fillers.
ADSCs: how stem cells may address the limitations of currently available fillers
For many years, the principal function of adipose tissue was thought to be energy and vitamin storage and secondarily, as a space filler serving to cushion the body’s internal organs and muscles. In the late 1990s cells within adipose tissue were isolated, characterized, and their differentiation capability into multiple cell phenotypes determined. This paved the path to our current understanding of the therapeutic potential of adipose tissue ( ). Similar to mesenchymal stem cells isolated from bone marrow, ADSCs demonstrated the ability to become extracellular matrix producing osteoblasts and chondrocytes, and more importantly for aesthetic purposes, into adipocytes equivalent to those that compromised the tissue from where they originated ( Figure 9.1 ). Subsequently, the cells were shown under different cell culture conditions to have the capacity to express smooth, skeletal and cardiac muscle phenotypes, and later, characteristics of neurons, glial, hepatocytic and vascular endothelial cells ( Figure 9.1D ) ( ).

In addition to their ability to undergo multi-potent differentiation, ADSCs were shown in vitro to express and secrete growth factors, cytokines, chemokines, and extracellular matrix molecules that are important for neovascularization, regulation of apoptosis, modulation of immune response and extracellular matrix remodeling and even chemokines important for the recruitment of endogenous stem and regenerative cells ( ). These data collectively support the hypothesis that ADSCs should be able to enhance revascularization, regulate matrix remodeling and thereby slow the rate of filler resorption, and perhaps, even maintain a self-renewing cell population of appropriately differentiated phenotype.
As pre-clinical studies of ADSCs were performed, two critical aspects of the nature of adult stem cells were realized. First, the environment into which ADSCs are introduced is a critical determinant of their functional efficacy. Second, their capacity to differentiate into the parenchymal cell phenotype of the tissue into which they are introduced does not appear to correlate to the functional improvement observed. While perhaps not surprising in hindsight, these observations have nevertheless established the major directions that ADSC therapy development continues to take.
There are several means by which ADSCs could be used for aesthetic repair or reconstruction. The most straightforward approach is to directly inject the ADSCs into the intra- or subcutaneous dermal space. In this scenario, ADSCs will either differentiate into dermal cells to generate more skin and associated extracellular matrix, or they may act on the endogenous cells, stimulating their proliferation and subsequent new tissue formation. Experimental evidence exists to support both possibilities ( , ). Technical issues concerning ADSC retention after injection and dependence on the presence of appropriate environmental cues within the treated site support the use of a modified version of this approach. Andrew Altman and colleagues ( ) recently reported results of human ADSC administration in an immunocompromised mouse dermal wound model. ADSCs transplanted into the wound site were combined with acellular dermal matrix (AlloDerm®).
Prior to transplantation ADSCs were genetically modified to express green fluorescent protein (GFP). Wound sites receiving the ADSCs healed significantly faster than the control wounds by one to two weeks after transplantation, and GFP positive ADSCs were found within the dermis. These cells were found to display either an epidermal or vascular endothelial phenotype. Thus, one advantage of the use of ADSCs as an aesthetic filler is their ability to differentiate into different cell types, generating not only the best natural filler (i.e. new skin and collagen matrix) but also contributing to the sustainability of the new tissue through enhanced vascularization.
An extension of this strategy is to develop combination fillers by injecting ADSCs along with currently available biocompatible fillers, especially those that are naturally present within the dermis, such as collagen. It is again important to emphasize that inappropriate environmental cues can have undesirable outcomes. Soft tissue fillers that contain hyaluronic acid, a well known matrix molecule within cartilage, could theoretically support chondrogenic differentiation of the ADSCs, and hydroxyapatite (calcium tri-phosphate) beads are commonly used in the orthopedic cell therapy field as a stem cell carrier for bone repair and have been shown to enhance bone regeneration.
Tissue engineering (TE) based strategies utilize ADSCs to generate new tissue ex vivo instead of depending on the cells to receive the appropriate cues to grow and maintain new tissue after in vivo injection. Several research groups have demonstrated the feasibility of seeding ADSCs onto natural or synthetic bio-polymer matrices to generate transplantable amounts of adipose tissue ( ). There are some significant technical and economic challenges to this approach. First, assuming the ADSCs are autologous, they must be obtained through a separate initial procedure, then isolated, expanded in vitro, harvested and either frozen until use or, alternatively, the secondary procedure must be coordinated with stem cell culture harvest. The manufacturing cost associated with TE based approaches for most aesthetic applications is unlikely to be competitive with other available fillers despite the potential benefits. However, if allogeneic ADSCs are used, both technical and cost issues could be reduced. A potential drawback to the use of allogeneic cells is that they may incur immuno-intolerance and rejection issues similar to those associated with other biochemically based fillers. Although current research data and supports allogeneic ADSCs as immuno-privileged and even immuno-modulatory cells similar to bone marrow derived mesenchymal stem cells, clinical safety of allogeneic ADSCs remain to be established across a large sampling of the human population ( ).
Another significant technical hurdle for tissue engineered ADSC fillers to reach the market is the need for rapid introduction of a vascular network within the graft. Unless methods of neovascularization are incorporated into the engineered grafts during their ex vivo culture, the grafts are subject to the same volume dependent oxygen and nutrient diffusion limitations that confound the survival of autologous fat graft alone. While neovascularization of AFG in preclinical models is observed to begin within 24 h of transplant, a week or longer may be required to sufficiently vascularize grafts used for large volume defects. During this period, necrosis within the inner regions of the graft could occur resulting in lipolytic cysts which are sometimes observed in large volume AFG procedures. Again, the economics of facial aesthetic procedures are unlikely to predicate the commercial development of pre-vascularized ADSC tissue engineered fat grafts in the near future.
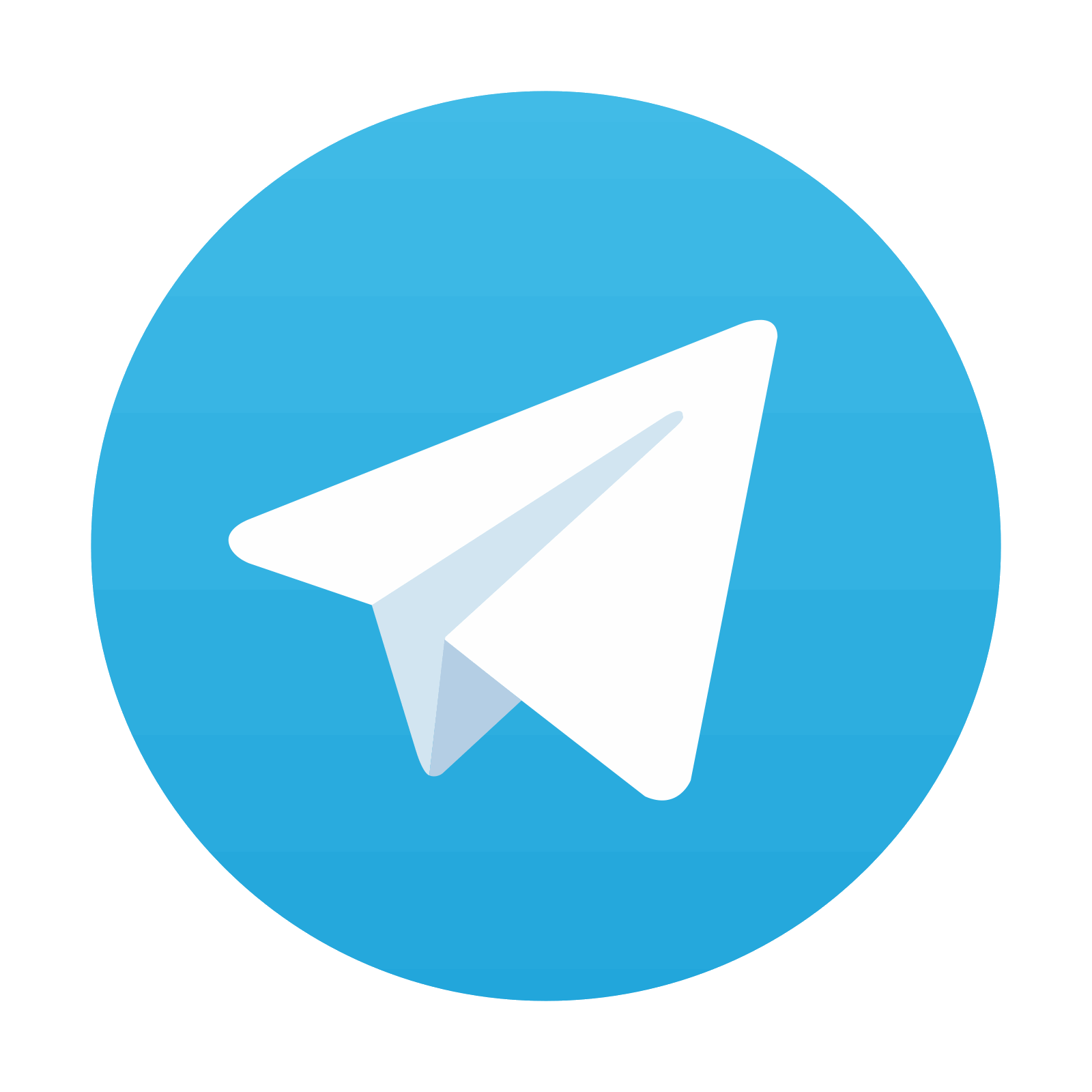
Stay updated, free articles. Join our Telegram channel
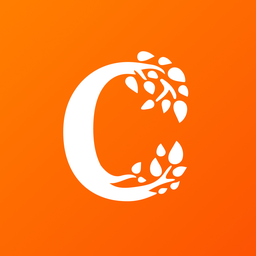
Full access? Get Clinical Tree
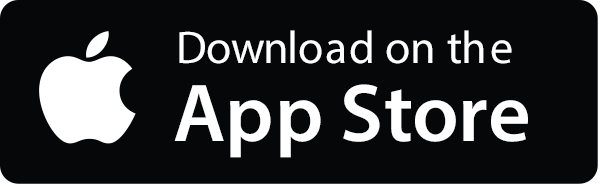
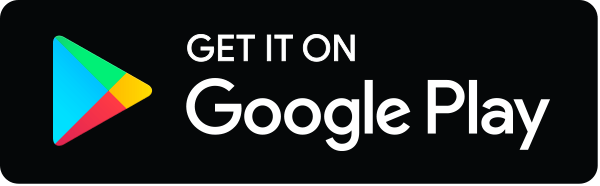