Changes in mechanical properties are an essential characteristic of the aging process of human skin. Previous studies attribute these changes predominantly to the altered collagen and elastin organization and density of the extracellular matrix. Here, we show that individual dermal fibroblasts also exhibit a significant increase in stiffness during aging in vivo. With the laser-based optical cell stretcher we examined the viscoelastic biomechanics of dermal fibroblasts isolated from 14 human donors aged 27 to 80. Increasing age was clearly accompanied by a stiffening of the investigated cells. We found that fibroblasts from old donors exhibited an increase in rigidity of ∼60% with respect to cells of the youngest donors. A FACS analysis of the content of the cytoskeletal polymers shows a shift from monomeric G-actin to polymerized, filamentous F-actin, but no significant changes in the vimentin and microtubule content. The rheological analysis of fibroblast-populated collagen gels demonstrates that cell stiffening directly results in altered viscoelastic properties of the collagen matrix. These results identify a new mechanism that may contribute to the age-related impairment of elastic properties in human skin. The altered mechanical behavior might influence cell functions involving the cytoskeleton, such as contractility, motility, and proliferation, which are essential for reorganization of the extracellular matrix.
Introduction
Aging is the result of a complex interaction of biological, physical, and biochemical processes that cause changes and damage to molecules, cellular function, and organs. To date, several studies have examined age-related alterations in cell division, biosynthesis, and cell migration, but there is little information about the relationship between aging and the mechanical properties of cells. Previous studies have shown that biomechanical properties are important for cell functions that are regulated by mechanical forces. Individual cells are able to sense mechanical signals and transduce them into a biochemical response. When the synthesis activity of cells is compared in stressed versus relaxed collagen gels, as well as for cells growing in collagen gels versus plastic dishes, a link is observed between the collagen production of fibroblasts and the mechanical forces acting on the cells. This is not surprising, since active and passive cellular biomechanics based on the stability provided by the cytoskeleton impacts many essential cellular functions. In addition to the role of the cytoskeleton in cellular motility and division, it has been shown that a functionally intact cytoskeleton is crucial to build up contraction forces in a three-dimensional collagen lattice. These cell functions are essential for the homeostasis of the extracellular matrix (ECM).
Considering the importance of cellular biomechanics for correct physiological functioning, we analyzed the mechanical behavior of dermal fibroblasts isolated from human donors of different ages. Changes in mechanical properties of the skin are generally referred to extracellular aspects such as alterations in polymerization and cross-linking of collagen and elastin. In this context, age-related changes have been observed for protein synthesis and production of ECM components. Further, atomic force microscopy studies indicate that individual epithelial cells show a considerable increase in stiffness in vitro at higher passage numbers. Even though comparisons of cells of early and late passage have yielded important insights into the aging process in vitro, such models cannot substitute for studies of cells isolated from aged human donors (aged in vivo). Thus far, no study has compared the viscoelastic properties of dermal fibroblasts from young and advanced-age donors. To address this issue, the optical stretcher technique was applied to examine the deformability of fibroblasts isolated from 14 human donors.
The microfluidic optical stretcher is an optical trap that allows trapping and controlled deformation of suspended cells using two counterpropagating laser beams. Cellular extension is caused by a momentum transfer acting on the cell surface. The global stress applied by the optical stretcher permits the measurement of whole-cell elasticity that characterizes the integral effects of molecular changes on the cytoskeleton. The use of optical deformability as a sensitive cell marker has already been demonstrated for characterization of individual cancer cell lines, as well as for cancer diagnosis by mechanical phenotyping of oral keratinocytes. We suggest that optical deformability can also serve as a sensitive biomarker of aging on the level of individual dermal cells. To date, cellular senescence as a measure of aging has been characterized by molecular markers based on an altered pattern of gene and protein expression. Typical known biomarkers are senescence-associated β -galactosidase, telomere dysfunction, expression of activated p53 and p16, and nuclear accumulation of globular actin.
Since viscoelastic properties are determined by the cytoskeletal polymers, and actin is supposed to be a key element, we further quantified the amount of polymerized actin using FACS measurements. In addition, possible age-related changes in vimentin and microtubule content were analyzed. In vivo, there are many cells able to perform mechanical work, for example, muscle cells or cells of connective tissues that are connected to the proteins of the ECM. Three-dimensional collagen lattices seeded with fibroblasts have been used to analyze the effect of actin or microtubule disruption on the mechanical activity of cells. The mechanical properties of cells and the state of the cytoskeleton affect the interaction between fibroblasts and the ECM, so mechanical changes may reveal new aspects of the age-related degradation of the elastic properties of the dermis. Rheological measurements were used to characterize fibroblast-populated collagen gels as dermis equivalents. These experiments determine the influence of changes in the mechanical properties of cells and the cytoskeleton on the organization and mechanical behavior of the collagen matrix.
Materials and methods
Cell Culture
Biopsies were isolated from plastic surgery procedures. We adhered to the recommendations of the current version of the Declaration of Helsinki as applied to a non-drug study. All donors provided written, informed consent. Human dermal fibroblasts (HDFs) were isolated by outgrowth from skin biopsy samples obtained from healthy female donors of different ages (20–80 years). Primary cells were enzymatically prepared using a dispase digestion technique. Square-cut biopsy pieces were washed, rinsed, and incubated in dispase (Boehringer Mannheim, Mannheim, Germany) for 2 h at 37°C and 7% CO 2 . The dermis and epidermis were then separated and the dermal fraction was cultured in six-well plates containing Dulbecco’s modified Eagle’s medium (Life Technologies, Eggenstein, Germany) supplemented with 10% fetal calf serum (Life Technologies) and penicillin/streptomycin (50 μ g/ml, Life Technologies). Confluent fibroblasts were seeded into appropriate flasks. For the experiments, cells were used at passage 3 grown to 90% confluency. Generally, the two age groups were defined as young donors <42 years and old donors >60 years. Due to limited biopsy material, donor ages can vary between different experiments.
Refractive Index Measurements
The refractive indices of fibroblast populations from different-aged donors were measured using a phase-matching technique. Cells are suspended in bovine serum albumin (BSA) phosphate-buffered saline solutions with different concentrations of BSA. Since the refractive index of the solution depends on the protein concentration, the refractive index of the surrounding medium is varied.
The exact refractive index of each solution is measured using an Abbe-refractometer (AR4, Krüss Optronics, Hamburg, Germany) and the appearance of the cells in a phase-contrast microscope is analyzed. Cells with an index of refraction lower than the surrounding medium are brighter than the background, and cells with a higher refractive index appear dark ( Fig. S8 A in the Supporting Material ). Assuming a normal distribution for the refractive indices of the cells, the percentage of cells that appear brighter than the background can be fitted by an error function ( Fig. S8 B):
Here, σ is the standard deviation of the distribution and μ is the mean value of the refractive index, which corresponds to the inflection point of the error function. Both parameters are determined by the fit of the data.
Optical Stretcher
The optical stretcher is an optical trap consisting of two coaxially aligned, counterpropagating divergent laser beams that apply optical forces to the surfaces of suspended cells. Increasing the laser power will deform the whole cell along the laser-beam axis. The stretching is caused by transfer of momentum to the cell membrane pointing away from the medium of higher refractive index. Stress magnitude and distribution can be calculated by a ray optics approach. A schematic of a resulting stress profile acting on the cell surface is shown in Fig. 1 A.
The setup consists of an ytterbium-doped fiber laser operating at a wavelength of 1064 nm (YLM-10-1064, IPG Photonics, Burbach, Germany) with a maximum output power of 10 W. The optical fiber was spliced to a 1 × 2 coupler, splitting the light in a 50:50 ratio. Cells were positioned between the two laser beams by a microfluidic delivery system For this, a glass capillary tube (VitroCom, Mountain Lakes, NJ) was situated between the two fiber ends ( Fig. 1 B). The optical fibers (PureMode HI 1060, Corning, Wiesbaden, Germany) were placed at a distance of ∼120 μ m from the capillary wall. The whole microfluidic system was mounted on an inverted phase-contrast microscope (DMIL, Leica, Solms, Germany) and deformations of the cells were recorded at a rate of 30 frames/s using a CCD camera (A202k, Basler, Ahrensburg, Germany). The images obtained were analyzed automatically by a LabView routine that determines the cell boundaries ( Fig. 1 A) and calculates the strain of the cells along the laser-beam axis. The laser power used was P = 0.2 W/fiber for trapping the cells and P = 1.2 W/fiber for the stretching. The resulting extension behavior was analyzed, and viscoelastic properties of the cells were calculated as described in Wottawah et al. In the three-parameter model used, optical deformability, which corresponds to the compliance of the cells, is described by
when the stress is applied (0 < t < t 1 ) and by
when the laser is off again ( t > t 1 ). Here, σ represents the applied stress and a 1 , a 2 , and b 1 are parameters derived from the viscoelastic constitutive equation. Further information about calculation of the stress, σ , can be found in Guck et al. and Wottawah et al. The constants can be obtained by fitting the data to allow the calculation of rheological parameters like the plateau Young’s modulus, E p , which is a measure of the overall elastic behavior of cells:
FACS Analysis
After incubation of fibroblasts to a confluence level of 90%, cells were trypsinized, centrifuged at 10,000 rpm, and washed twice with PBS. Cells were then resuspended in 3% paraformaldehyde and incubated for 30 min at room temperature. After two additional washing steps, the cells were permeabilized with 0.5% Triton-X100 (Sigma, St. Louis, MO) in PBS. Cells were then washed, blocked for 30 min with 3% BSA, and washed again. For actin staining, Alexa-488-labeled Dnase I and Alexa-647-labeled phalloidin (Invitrogen, Paisley, United Kingdom) were added at concentrations of 161.0 μ M and 6.6 μ M, respectively. Cells were incubated for 20 min at room temperature. After two washing steps with PBS, the fluorescence was measured using a FACSCanto flow cytometer (BD Biosciences, San Jose, CA) and analyzed by the software BD FACSDiva 4.0.
Concerning microtubule staining, the cells were incubated for 30 min with Oregon Green 488 conjugated paclitaxel (Invitrogen) at a concentration of 1.0 μ M. Vimentin was detected using an antibody (sc-32322, dilution 1:200) purchased from Santa Cruz Biotechnology (Santa Cruz, CA).
Tissue Rheology
For preparation of dermis equivalents, 100 mg Type I collagen (Sigma, St. Louis, MO) was dissolved at 4°C in 33.3 ml of 0.1% sterile acetic acid (c = 3 mg/ml). The used fibroblast populated gels consist of 80% collagen solution, 10% 10× Hanks’ buffer salt solution (Biochrom, Berlin, Germany), and 10% fetal calf serum containing 3 × 10 6 suspended cells/ml. The final solution contained 2.4 mg/ml collagen and 3 × 10 5 fibroblasts/ml. NaOH (1 M) was added dropwise to neutralize the solution, whereof 2.5 ml was applied to six-well plates (Greiner, Germany) and incubated at 37°C and 7% CO 2 for 1 h. The gels were covered with 2 ml medium (DMEM, 1% penicillin/streptomycin, 1% glutamax, and 10% FCS) and incubated for another 23 h at 37°C and 7% CO 2 . To study the specific effect of cytoskeletal stiffening, collagen gels were treated for 48 h with 0.5 μ M jasplakinolide in DMEM. Jasplakinolide is described as promoting actin polymerization and stabilizes actin filaments, leading to increased cellular stiffness. A possible connection between actin stabilization and cellular aging has already been hypothesized by Gourlay et al. who measured an increased reactive oxygen species production in connection with decreased actin turnover induced by jasplakinolide. Although the effect of jasplakinolide treatment is controversial in literature, optical stretcher experiments confirmed a higher cell stiffness after drug treatment ( Fig. S10 ).
Viscoelastic properties of the gels were measured by a shear rheometer (AR2000ex, TA Instruments, New Castle, DE) using a plate-plate geometry (diameter, 2 cm). The AR2000ex offers different modes of oscillatory experiments to determine the complex shear modulus, G ∗ = G′ + iG″, which depends on shear frequency, stress, and strain. Fibroblast-populated collagen gels were characterized by measurement of the elastic storage modulus, G′, and the viscous loss modulus, G″, in the linear viscoelastic regime ( γ = 2% and ω = 5 rad/s). At higher deformation, plastic effects were observed in the form of decreasing storage moduli.
Materials and methods
Cell Culture
Biopsies were isolated from plastic surgery procedures. We adhered to the recommendations of the current version of the Declaration of Helsinki as applied to a non-drug study. All donors provided written, informed consent. Human dermal fibroblasts (HDFs) were isolated by outgrowth from skin biopsy samples obtained from healthy female donors of different ages (20–80 years). Primary cells were enzymatically prepared using a dispase digestion technique. Square-cut biopsy pieces were washed, rinsed, and incubated in dispase (Boehringer Mannheim, Mannheim, Germany) for 2 h at 37°C and 7% CO 2 . The dermis and epidermis were then separated and the dermal fraction was cultured in six-well plates containing Dulbecco’s modified Eagle’s medium (Life Technologies, Eggenstein, Germany) supplemented with 10% fetal calf serum (Life Technologies) and penicillin/streptomycin (50 μ g/ml, Life Technologies). Confluent fibroblasts were seeded into appropriate flasks. For the experiments, cells were used at passage 3 grown to 90% confluency. Generally, the two age groups were defined as young donors <42 years and old donors >60 years. Due to limited biopsy material, donor ages can vary between different experiments.
Refractive Index Measurements
The refractive indices of fibroblast populations from different-aged donors were measured using a phase-matching technique. Cells are suspended in bovine serum albumin (BSA) phosphate-buffered saline solutions with different concentrations of BSA. Since the refractive index of the solution depends on the protein concentration, the refractive index of the surrounding medium is varied.
The exact refractive index of each solution is measured using an Abbe-refractometer (AR4, Krüss Optronics, Hamburg, Germany) and the appearance of the cells in a phase-contrast microscope is analyzed. Cells with an index of refraction lower than the surrounding medium are brighter than the background, and cells with a higher refractive index appear dark ( Fig. S8 A in the Supporting Material ). Assuming a normal distribution for the refractive indices of the cells, the percentage of cells that appear brighter than the background can be fitted by an error function ( Fig. S8 B):
Here, σ is the standard deviation of the distribution and μ is the mean value of the refractive index, which corresponds to the inflection point of the error function. Both parameters are determined by the fit of the data.
Optical Stretcher
The optical stretcher is an optical trap consisting of two coaxially aligned, counterpropagating divergent laser beams that apply optical forces to the surfaces of suspended cells. Increasing the laser power will deform the whole cell along the laser-beam axis. The stretching is caused by transfer of momentum to the cell membrane pointing away from the medium of higher refractive index. Stress magnitude and distribution can be calculated by a ray optics approach. A schematic of a resulting stress profile acting on the cell surface is shown in Fig. 1 A.
The setup consists of an ytterbium-doped fiber laser operating at a wavelength of 1064 nm (YLM-10-1064, IPG Photonics, Burbach, Germany) with a maximum output power of 10 W. The optical fiber was spliced to a 1 × 2 coupler, splitting the light in a 50:50 ratio. Cells were positioned between the two laser beams by a microfluidic delivery system For this, a glass capillary tube (VitroCom, Mountain Lakes, NJ) was situated between the two fiber ends ( Fig. 1 B). The optical fibers (PureMode HI 1060, Corning, Wiesbaden, Germany) were placed at a distance of ∼120 μ m from the capillary wall. The whole microfluidic system was mounted on an inverted phase-contrast microscope (DMIL, Leica, Solms, Germany) and deformations of the cells were recorded at a rate of 30 frames/s using a CCD camera (A202k, Basler, Ahrensburg, Germany). The images obtained were analyzed automatically by a LabView routine that determines the cell boundaries ( Fig. 1 A) and calculates the strain of the cells along the laser-beam axis. The laser power used was P = 0.2 W/fiber for trapping the cells and P = 1.2 W/fiber for the stretching. The resulting extension behavior was analyzed, and viscoelastic properties of the cells were calculated as described in Wottawah et al. In the three-parameter model used, optical deformability, which corresponds to the compliance of the cells, is described by
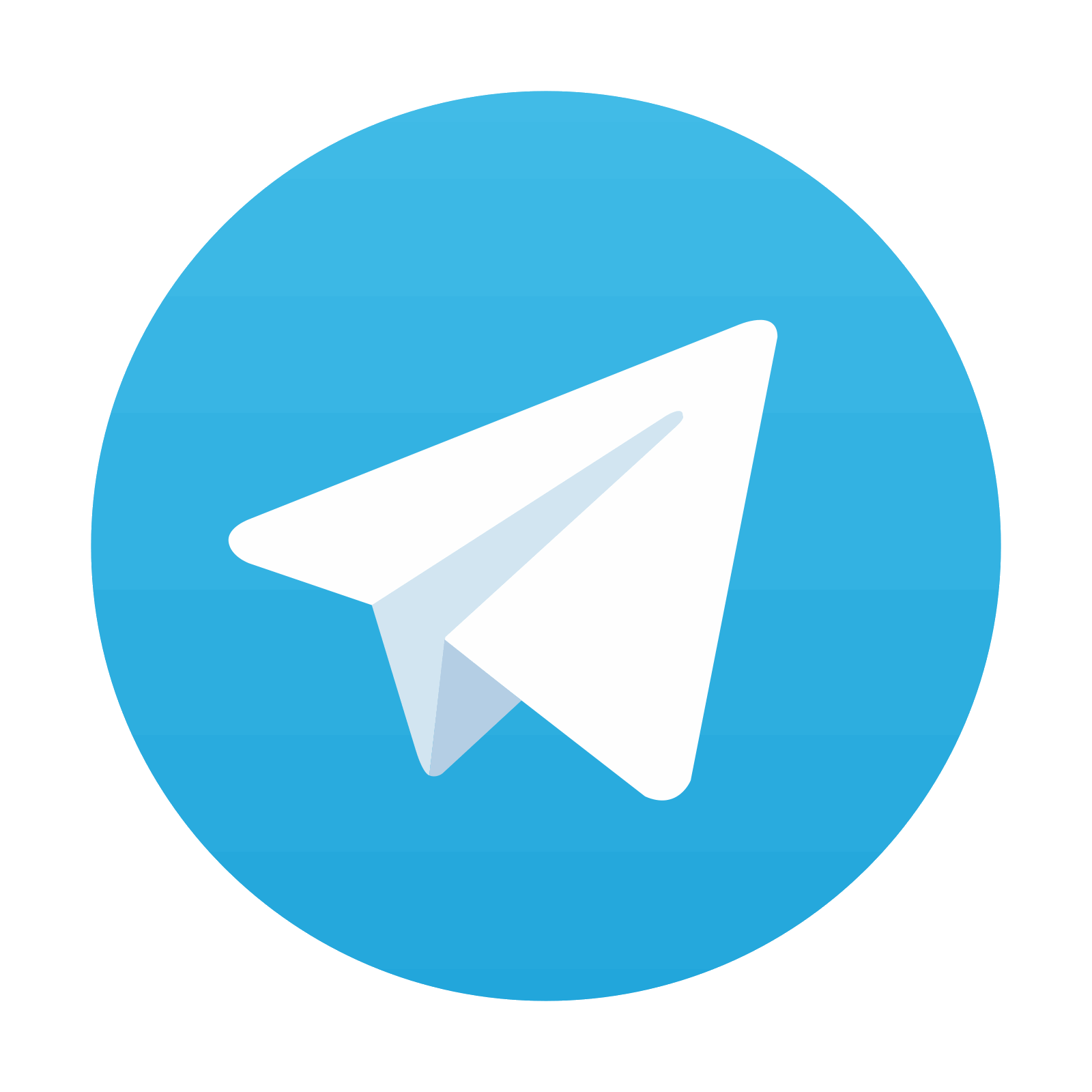
Stay updated, free articles. Join our Telegram channel
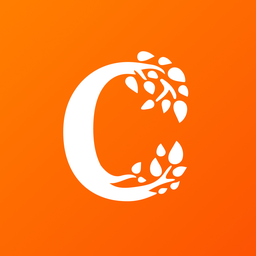
Full access? Get Clinical Tree
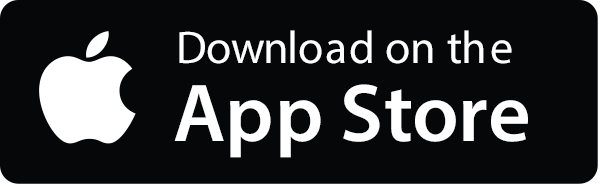
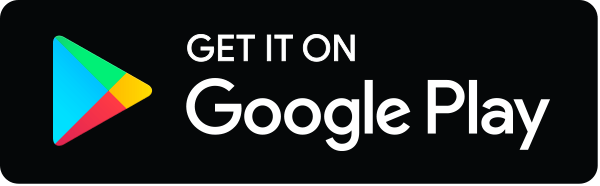