13 Stem cells and regenerative medicine
Synopsis
There is a significant biomedical need for tissue reconstruction and regeneration.
Stem cells are a key building block to any tissue regeneration or engineering strategy.
Stem cells can be derived from multiple sources and tissues which exist at different stages of differentiation.
Stem cells can have different in vitro and in vivo capabilities and are at a different stage in bench and bedside research:
Prospective clinical applications for stem cell therapy will likely require multidisciplinary approaches employing tissue engineering and inductive therapies (biomimetic matrices, gene therapy, small molecules, growth factors).
Introduction
• Three characteristics that define a stem cell are:
• Stem cells can come from embryonic or postnatal sources.
• Embryonic stem cells exist in a more pluripotent state than postnatal, somatic, and tissue-specific stem cells.
• MSC differentiation has been elucidated using specific in vitro and in vivo protocols.
• Adult, or tissue-specific, stem cells are undifferentiated cells which are found in almost all tissues and organs after embryonic development.
• Postnatal stem cells can be reprogrammed into an “embryonic-like” state called induced pluripotent cells.
Discussion of biomedical burden (Fig. 13.1)
As the global population continues both to age and expand, so does the associated incidence of diseases, defects, and deficits afflicting patients secondary to a multitude of congenital, postsurgical, posttraumatic, vascular, and degenerative etiologies (arthritis, osteoporosis, chronic wounds, postoncologic resection). In 2007, it was estimated that diseases of the musculoskeletal system cost over 26 billion dollars in the US with an average annual growth of 8.5% (second highest growth of all body systems).1 With regard to cancer reconstructions, the American Society of Plastic Surgery estimates that almost 5.2 million reconstructive procedures were performed by plastic surgeons in 2009, over 3.9 million for cancer reconstruction, and over 86 000 breast reconstructive cases.2
Thus plastic surgeons struggle due to the inability of current implants and grafts to replicate completely the ability of a dynamic living tissue to remodel and regenerate in response to environmental cues. Ultimately reconstructive strategies must shift from simple tissue repair to tissue regeneration. The fundamental idea behind tissue regeneration is to use a biologically active scaffold seeded with a pluripotent, self-renewing cell type. The synergy of a biomimetic scaffold with a competent cellular element will allow creation of tissues that are able to respond and regenerate in the face of injury. Such cells exist in abundance from embryos to the elderly, namely, stem cells (Fig. 13.2).
Stem cells are defined functionally as a clonogenic population of cells capable of self-renewal and differentiation to committed progenitors and subsequently to differentiated, functional tissues.3 Traditionally, stem cells have been divided into two categories based on their ability to differentiate. Pluripotent stem cells (embryonic) can differentiate into any cell type in the body, whereas multipotent stem cells (adult) can differentiate into multiple, but not all, cell lineages. In addition to the traditional stem cell classification, a new class of stem cells has recently been described – induced pluripotent stem (iPS) cells – which are derived from genetically reprogrammed adult cells that are believed to have the potential of embryonic stem cells.
Why stem cells and regenerative medicine should be of interest to plastic surgeons
Plastic surgeons stand at the clinical forefront of using ASCs as they have direct access to large quantities of adipose tissue from liposuction as well as body-contouring procedures. The hope of clinicians would be one day to take a patient to the operating room, and in one stage, use a prefabricated biomimetic scaffold construct and seed this with stem cells derived from a small-volume lipoaspirate to address a soft-tissue, skeletal, muscle, cartilage, vascular, or joint defect. Furthermore, as orthopedic surgeons increase their volume in spine surgery and bone morphogenic protein-2 (BMP-2) usage, we foresee plastic surgeons helping with immediate liposuction and ASC harvest in conjunction with growth factors such as BMP-2 for bony regeneration. Recent studies have shown that there may be differences in osteogenic and adipogenic potential in the subcutaneous region from which the cells are harvested and thus surgeons may tailor the region from which they harvest fat to address patient needs best.4 The potential breakthroughs in the use of human stem and stromal cells have prompted some individuals to store their own tissues in a fee-for-service fashion. The most obvious example is the cryostorage of umbilical cord-derived blood.5 The process of cell freezing and long-term storage have been applied to human ASCs (hASCs) as well.
Historical perspective
Current concepts of a clonal stem cell population have only been validated in the last 40 years. Prior to the 1960s all dividing cells were thought to contribute to tissue growth and turnover and were considered resident stem cells.3 A revolutionizing publication in 1961 defined the method for isolating a population of self-renewing cells in the bone marrow.6 This initial discovery was found by infusing a lethally irradiated mouse with bone marrow cells that would migrate to the spleen and give rise to hematopoietic cell nodules that were proven to derive from the same single cell or clone. These clones were named “colony-forming units” and eventually were shown to be short-term hematopoietic cells. Such studies provided the groundwork for the understanding of the existence of an undifferentiated self-renewing cell population.
The presence of MSCs in the bone marrow was first suggested in the late 19th century by Cohneim, who proposed that fibroblasts involved in peripheral wound healing were derived from the marrow compartment.7 In the 1970s, Friedenstein et al. demonstrated the existence of “fibroblastoid” cells within guinea-pig bone marrow by flushing out cells on to plastic culture dishes and discarding nonadherent cells.8 The remaining spindle-like cells were heterogeneous in appearance but capable of forming colonies in vitro, hence the name colony-forming unit fibroblasts.8,9 When these cells were subsequently transplanted into subcutaneous pockets, they were found to form heterotopic bone tissue.8 Using similar methods, Castro-Malaspina et al. were able to isolate colony-forming unit fibroblasts from human bone marrow.10 Since these early studies, subsequent investigations have shown that these cells could be subpassaged in vitro and differentiated into several cell types of mesenchymal lineage.11,12 What Friedenstein had thus isolated would later be more aptly renamed by Caplan as “mesenchymal stem cells.”11
The fundamental characterization of MSCs as truly multipotent cells can be attributed to work by Pittenger and colleagues in 1999.13 Prior to this report, the question remained whether bone marrow MSCs represented mixtures of committed progenitor cells, each with restricted potential, or individual cells capable of differentiating into fat, cartilage, bone, and muscle. Using human bone marrow aspirates from the iliac crest of over 350 donors and a density gradient isolation procedure, a phenotypically homogeneous population of adherent cells was obtained with over 98% of cells sharing the same surface marker profile by flow cytometric analysis. Single cells were isolated and re-expanded, and then shown to retain their differentiation capacity into adipocytes, chondrocytes, and osteoblasts.13 Therefore, MSCs were proven to have the ability to proliferate extensively while maintaining their multipotent nature, putatively establishing these cells as true stem cells.
Historically, adipose tissues were presumed to be a metabolic reserve playing a key role in processing, storing, and liberating high-energy materials in the form of cholesterol and triglycerides. Similar to bone marrow, scientists in the early 1960s described a stromovascular fraction (SVF) that contained fibroblastic-like cells.14 Subsequent research of this SVF cell type led scientists to believe that they represented an adipose progenitor cell whose fate was limited to adipose tissue.15 In 2001, however, the understanding of the potential of these SVF cells greatly changed as Zuk et al. demonstrated the ability of these cells to undergo not only adipogenic differentiation but also chondrogenic, myogenic, and osteogenic differentiation.16 These cells were eventually renamed adipose-derived stromal cells or ASCs. Similar to MSCs, ASCs have also been shown to differentiate into cardiac cells and even neural progenitor cells in vitro.16–18 The multipotency of ASCs has grown tremendously since these early studies as scientists continue to fine-tune and improve the differentiation of each pathway.17,19–24 Cell surface markers on ASCs have been shown to be similar to those of MSCs as both express CD105, STRO-1, CD29, CD144, and CD166.24,25 Adipose tissue-derived stem cells lack the expression of known hematopoietic and endothelial markers such as CD3, CD4, CD11c, CD14, CD15, CD16, CD19, CD31, CD33, CD38, CD56, CD62p, CD104, and CD144. Due to their significant availability and ease of harvest, ASCs have become a common topic of tissue-engineering research. For example, numerous studies have demonstrated the potential of ASCs seeded on to a polyglycolic scaffold to create an osteoid-like material.26–28
Establishment of embryonic stem cells was first reported in 1981 using preimplantation mouse embryos.29 Cells from blastocysts were explanted into tissue culture and four individual cell lines were developed. When transplanted into syngeneic murine hosts, formation of well-differentiated teratocarcinomas was observed, suggesting that these embryonic cells possess pluripotent capacity.30 Perhaps the most pre-eminent consideration encompassing early research with human embryonic stem cells (hESCs) has been the contentious political and ethical debate surrounding creation, usage, and destruction of human embryos. Until recently, the creation of hESCs necessitated destruction of a human embryo which naturally raised various value-of-life objections. Many of these embryos, however, were created but not used for in vitro fertilization and had been destined for destruction after storage periods long past their viable shelf-life.31 In 2009, President Obama expanded federal funding beyond what had been previously authorized to include more than 100 existing cell lines, in addition to newly created hESCs sponsored by private or state-level funding.32 Under this new provision, federal funds could still not be awarded to create new stem cell lines involving destruction of an embryo. As a means potentially to sidestep this restriction, a novel method of hESC derivation was described that did not interfere with the embryo’s developmental potential.33 Using a single-cell biopsy technique similar to that used in preimplantation genetic diagnosis, researchers were able to generate two new pluripotent cell lines without destroying the embryo.33 This approach would also allow for potential generation of matched tissue for children born through in vitro fertilization.
An important concept in adult stem cell function is the stem cell niche, first proposed by Schofield in 1978.34 It describes the microenvironment of cells and signals which permit adult stem cells to maintain their function as regenerative cells.35 This structural unit critically modulates the surrounding stimuli to sustain adult stem cell populations while also ensuring that they are appropriately activated. Studies in hair follicle, intestinal lining, and bone marrow have revealed significant insight into the precise spatiotemporal regulation of this interactive crosstalk which is conserved across species and biologic systems.36 Thus, significant advancements have been made with regard to stem cells and stem cell biology from pluripotent to unipotent cell types. As plastic surgeons, we can learn from this history and apply our unique knowledge of surgical reconstruction to bring these benchtop findings to the bedside.
Human embryonic stem cells
Definitions
Research in hESCs has been a rapidly developing field, attracting increasing attention over the last decade. The capacity of hESCs to reproduce almost any cell type found in the human body has led to many promising areas of investigation which may yield a deeper understanding of cellular biology and potential cures for many diseases.37,38 In addition, the ability to repair and regenerate tissues injured by trauma may facilitate the development and implementation of new treatment paradigms employing hESCs to restore areas of organ damage and reverse what was once thought to be permanent functional deficit. Despite recent controversy surrounding political and ethical concerns over the research and clinical use of these cells, studies continue with hESCs and considerable promise still remains for this area of investigation.
The term “embryonic stem cell” was coined by Gail Martin to distinguish them from previously described pluripotent embryonal carcinoma (EC) cells derived from teratocarcinomas.29 At root, ESCs possess three essential characteristics: (1) derivation from a preimplantation or peri-implantation embryo; (2) the capacity to self-renew and proliferate in a prolonged undifferentiated state; and (3) the ability to form derivates of all three embryonic germ layers – or pluripotency – after prolonged culture.39
As an extension of this early work in mice, hESCs were first isolated in 1998.39 Donated fresh cleavage-stage human embryos produced by in vitro fertilization were obtained by Thomson et al. and cultured to the blastocyst stage, typically reached at 4–5 days postfertilization. From the inner cell mass (which ultimately forms the embryo), five separate hESC lines were established and successfully maintained in culture for 6 months in an undifferentiated state. All five lines also retained the capacity to form teratomas after injection into immunodeficient mice. Histological examination of these teratomas revealed gut epithelium, cartilage, bone, smooth muscle, neural epithelium, ganglia, and stratified squamous epithelium. Similar findings were also described by Reubinoff and colleagues, who derived two additional hESC cell lines and demonstrated expression of the transcription factor Oct-4 in these cells which had previously been shown to be essential for the maintenance of pluripotential capacity in mouse ESCs.40,41
With the development of hESCs, enthusiasm flourished surrounding their potential as experimental platforms to study a multitude of disease states. Studies designed to provide insight into human embryogenesis, development of birth defects, and cellular mechanisms of a variety of pathologic states proceeded at a feverish pace. But much of this early scientific fervor was tempered by several significant concerns. In theory, hESC possess the capacity to treat a wide variety of genetic diseases, cancers, diabetes, neurologic degenerative conditions, and spinal cord injuries. Use in such a clinical capacity, however, raises the important issue of graft-versus-host disease associated with allogeneic stem cell transplantation. One solution proffered for this histoincompatibility centers around the development of several hESC cell lines from variegated genetic backgrounds for tailored use in patients to minimize risk of rejection. Other strategies proposed include the use of autologous donor adult stem cells or the more recently described iPS cell.13,18,42
While work continues on reduction of donor host rejection, concerns regarding xenogeneic contamination have also been raised. Since their first isolation, hESCs have been traditionally cultured in vitro in the presence of mouse embryonic fibroblast (MEF) feeder layers.39 In their absence, hESCs have been found to undergo rapid differentiation. A feeder-free culture system was presented by Xu and colleagues employing Matrigel in medium conditioned by MEFs, but in both techniques, hESCs were exposed to murine products to maintain their pluripotency.43 In 2005, Martin et al. reported the presence of a nonhuman sialic acid Neu5Gc on the cell surface of hESCs.44 As humans are unable to generate this particular sialic acid, this likely represented uptake from media containing animal products and incorporation through the process of glycosylation. When these hESCs and the embryoid bodies they formed were exposed to human serum, rapid binding of immunoglobulin and deposition of complement were noted, ultimately resulting in cellular death.
Circumventing this problem necessitated the creation of a new stem cell line under murine-free conditions. To accomplish this, novel extracellular matrix-coated plates were developed from MEFs and sterilized prior to use.45 When hESCs were cultured using these plates, undifferentiated proliferation was observed for 6 months and cells maintained the capacity to form all three embryonic germ layers. This system thus eliminated exposure of hESCs to potential contamination from serum and/or live feeder cells and minimized the risk of disease transmission through contact of cells with animal or human pathogenic agents.
Current concepts and research
Scientific investigation with hESCs has begun to reveal the potential of these cells to revamp our contemporary understanding and treatment of disease dramatically (Fig. 13.3). Technologies which may be derived from stem cell research may be used one day to manage spinal cord injuries, degenerative neurologic conditions, and a variety of genetic diseases. While treatment of spinal cord trauma and Parkinson’s disease has received more high-profile attention of late, stem cell investigators have also made significant strides in the fields of diabetes, cardiac, and hematopoietic/vascular research.
The ability for ESCs to undergo neuronal differentiation was first demonstrated by Bain and colleagues in 1995.46 Exposing mouse ESCs to retinoic acid, multiple cellular phenotypes were observed, a large percentage of which produced neuron-like outgrowths. Gene expression analysis of these cells revealed several neural-associated transcripts including neurofilaments, glutamate receptor subunits, and nerve specific transcription factors. Furthermore, physiologic studies revealed these neuron-like cells could generate action potentials. Paralleling these experiments, Schuldiner et al. induced neuronal differentiation from hESCs using both retinoic acid and nerve growth factor (NGF).47 These neural progenitors were found to be capable of differentiation in vitro into astrocytes, oligodendrocytes, and mature neurons.40,48 When transplanted into the ventricles of newborn mouse brains, these hESC-derived neuronal cells could be observed to distribute widely throughout the brain and integrate in a region-specific manner. These findings therefore reveal the developmental potential of hESCs and their capacity to respond in vivo to local environmental cues to differentiate into appropriate neural lineages. While only preliminary, such findings also shed hope for the use of hESCs in the future treatment of neurological disease.
Similar to neural stem cell research, pluripotent hESCs have likewise shown promise in the field of diabetes research. At present, the only therapy considered potentially curative for type 1 diabetes is pancreatic islet cell replacement.49 Unfortunately, donor shortage has severely limited this modality from becoming a practical therapeutic solution. Furthermore, the risk of potential disease transmission and cellular rejection remains exigent. The discovery of glucose-sensitive insulin-secreting cells derived from mouse ESCs, however, has introduced a new potential source of cells for treatment of type 1 diabetes.49 Culturing hESCs in suspension and allowing for embryoid body formation also led to the detection of insulin-producing cells after 14 days of differentiation.50 By immunohistochemical staining, 1–3% of all cells were found to stain positively for insulin and were noted to be interspersed among the mixed population of spontaneously differentiating cells. More recent studies have cast some doubt on the frequency of these cells, though, estimating their true incidence to be less than 1 per 100 000 cells.51 Continued work must be performed, but these studies nonetheless demonstrate the potential for development of cells capable of β-islet function and insulin release. Such a subset of cells derived from hESCs could theoretically be exploited as a source of cell replacement for treatment of patients with type 1 diabetes.
The study of cardiac tissue development has long been handicapped by the lack of a suitable in vitro model. The advent of hESC research, however, has also offered a potential avenue for progress to be made in this field. Kehat and colleagues first noted the presence of spontaneously contracting areas within hESCs cultured in suspension and then plated to form embryoid bodies.52 Cells from these regions, which comprised approximately 8% of the entire area, stained positively for a variety of cardiac markers, including myosin, desmin, tropinin I, and atrial natriuretic factor. In addition, both positive and negative chronotropic effects were observed with these contracting cells following application of isoproterenol or carbamylcholine, respectively. Differentiation of hESCs into these cells could also be enhanced by the application of 5-aza-2’-deoxycytidine and purified through density centrifugation to obtain a population of cells containing 70% cardiomyocyte progenitors.53
In 2003, Mummery et al. provided the first demonstration of hESC cardiomyocyte differentiation through co-culture techniques with visceral endoderm-like cells.54 This approach mitigated the need for spontaneous cardiogenesis and efficiently produced solid aggregates of beating cells consisting of 10–200 cardiomyocytes. Interestingly, these colonies could also be frozen and were observed to resume beating upon thawing. Collectively, the findings presented strongly argue for continued development of hESCs as a model for cardiac research. Their ability to differentiate into cardiomyocytes and potential capability to enrich for these cells naturally lend toward the future development of clinical applications in heart disease.
In concert with work on cardiac differentiation, investigators have also discovered the capacity for hESCs to form vascular endothelial cells. Endothelial cells are critical for tissue repair and regeneration and the potential for hESC-derived vascular cells to participate in treatment of vascular disease is promising.55 Zambidis et al. first described the presence of mesodermal-hematoendothelial cluster colonies within embryoid bodies at days 6–9.56 These colonies were found to consist of nonadherent cells expressing CD45, which gave rise to hematopoietic lineages, and adherent CD31 cells which expressed markers characteristic for vascular endothelium. Isolation of these hESC-derived endothelial cells was accomplished through flow cytometry using anti-CD31 antibodies.57 These cells could then be expanded in culture and, when injected into immunocompromised mice, they were shown to form microvascular networks. While their long-term stability is unknown, they represent the first step towards potential treatment of vascular disease and stimulation of ischemic tissue growth using hESCs. They also provide a foundation for future study of the biomolecular mechanisms involved in blood vessel formation.
Clinical correlates
The capacity for regeneration of the central nervous system is limited, making the potential for repair and return of function through hESC therapy compelling. Following trauma, injuries to the brain and spinal cord may result in demyelination secondary to death of local oligodendrocytes.58 While some axons may be spared, action potential propagation may become disrupted, resulting in irreversible loss of motor function. The ability for hESCs to be differentiated into oligodendrocytes, however, offers some optimism for clinical recovery. As a proof of this concept, Keirstead and colleagues evaluated the ability for hESCs to incorporate, remyelinate, and restore locomotion after spinal cord injury in rats.59 Spinal cord contusion injuries were induced in Sprague–Dawley rats at the T10 level using a controlled, reproducible force generator capable of delivering a sudden desired impact. Oligodendryocytes derived from hESCs were purified and delivered both above and below the place of contusion 7 days following injury through direct injection into the spinal cord. Histological analysis 8 weeks later revealed significantly greater density of remyelinated axons when compared to control animals. More importantly, however, animals that received hESC-derived oligodendrocytes demonstrated significantly higher locomotor function scores which continued to improve up to 1 month after initial injury. These findings therefore revealed that transplantation of hESCs differentiated into oligodendrocytes may be an effective means of treating acute spinal cord injuries. Of note, no teratomas were observed in this study, suggesting predifferentiation of hESCs prior to clinical use may obviate concerns surrounding this potential complication.60
Following these promising data presented in rats for functional recovery, the biotechnology firm Geron petitioned for FDA approval to proceed with human trials in which hESCs would be similarly used to treat patients with spinal cord injuries. A phase I trial for this work was granted in January 2009, allowing for 8–10 patients with severe spinal cord injuries to be treated with hESC-derived oligodendrocytes. However, this trial has been put on hold by the FDA pending further review of preclinical data. While it remains to be seen if the findings described in rats can be clinically replicated, this represents the first step towards future translational therapies designed to incorporate these cells to treat human disease. Though full restoration of function following complete paralysis is not expected at this present time, there is hope that patients with less severe injuries may one day benefit from use of hESCs.61
Postnatal and somatic stem cells
Adipose-derived stromal cells
Definitions and harvest
Adipose tissue contains a stromal population that consists of microvascular endothelial cells, smooth-muscle cells, and multipotent cells. ASCs display the capacity to differentiate into adipocytes, osteoblasts, and chondroblasts in vitro.16,18,19,62,63 However, the origin of hASCs has not been clearly defined. Several laboratories have hypothesized that these cells represent pericytes surrounding blood vessels, which may explain their ability to differentiate into endothelial cells; others suspect they are a subpopulation of fibroblasts that reside within adipose tissue (Fig. 13.4).
ASCs, like stem cells from the bone marrow, have an extensive proliferative potential and can self-renew as well as undergo osteogenic, chondrogenic, myogenic, and adipogenic differentiation. This population can also be defined by its cell surface markers which have been shown to be positive for CD105, STRO-1, CD29, CD144, and CD166 and negative for CD3, CD4, CD11c, CD14, CD15, CD16, CD19, CD31, CD33, CD38, CD56, CD62p, CD104, and CD144.24,25
ASC harvest
Human ASC harvest can be performed on human lipoaspirate or resected adipose tissue. (Fig. 13.5). The benefit of using lipoaspirate is that it removes the step of having to mince the adipose tissue finely. Furthermore, it has been shown that, even following ultrasonic-assisted liposuction, the ASCs retain their ability to undergo osteogenic differentiation.64 If fat has been harvested from multiple anatomic locations, these specimens should be kept separate and labeled as differences exist in the differentiative capacity of different subcutaneous depots4 (Fig. 13.6). Following lipoaspiration, the adipose tissue should be processed as soon as possible in a sterile cell culture environment (Fig. 13.7). Adipose specimens should first be washed in dilute Betadine, followed by two phosphate-buffered saline (PBS) washes of equal volume to each lipoaspiration specimen. Tissues should then be subsequently digested with an equal volume of 0.075% (w/v) type II collagenase in Hank’s balanced salt solution at 37°C in a water bath with agitation at 140 rpm for 60 minutes. Every 15 minutes during digestion, the fat should be agitated and vented. Next, the collagenase digest should be inactivated by adding an equal volume of PBS with 10% fetal bovine serum (FBS) and 1% Pen/Strep. The stromal vascular fraction is then pelleted via centrifugation at 1000 rpm for 6 minutes. The supernatant is discarded and the cell pellet resuspended and filtered through a 100-µm cell strainer to remove undigested tissue fragments. The cells are pelleted and resuspended in growth media, and primary culture is established in tissue culture plates incubated at 37°C in an atmosphere of 5% CO2. Cell counting can be challenging given the large number of erythrocytes in the final cell pellet; however, we do not recommend a red cell lysis step as we believe this decreases the viability of the ASCs. On average, 10 mL of starting adipose tissue will allow the harvest of 1 million hASCs.
Current concepts and research
Difference between mouse and human ASCs
The study of mASCs is attractive due to the relative ease of cell harvest and the availability of laboratory mice. Moreover, the widespread use of genetic knockout mice makes for potential profitable avenues of investigation. However, there exist important differences between ASCs of mouse and human origin, differences which have not yet been fully investigated. For example, hASCs have been observed to have a significantly more robust in vitro osteogenic capacity as compared to mASCs.65,66 In order to undergo significant in vitro osteogenesis, mASCs require an additional osteogenic stimulus such as retinoic acid.67 Moreover, cytokines such as fibroblast growth factor (FGF)-2 abolish osteogenic differentiation in mASCs, whereas hASC osteogenic differentiation proceeds relatively uninhibited in the presence or absence of FGF-2.66,68
In our laboratory, we have observed that ASCs, whether derived from mouse or human origin, contribute to osseous healing of mouse cranial defects.69,70 For example, a critical-sized mouse calvarial defect shows no healing without ASC engraftment even up to 16 weeks postinjury. Upon hASC engraftment, however, significant bony healing is observed in as little as 4 weeks postinjury.70
Enrichment based on cell surface receptors
While substantial progress has been made in the study of ASCs, we are limited by working with a heterogeneous cell population. We believe that a selected, or clonal, subpopulation of cells enriched based on their cell surface markers is necessary to move our investigations forward (Fig. 13.8). By optimizing and selecting for an enriched subpopulation of ASCs that demonstrates a robust ability to differentiate along an osteogenic lineage, we can maximize the outcomes of cell-based therapies for skeletal regeneration. Borrowing an approach used to isolate hematopoietic stem cells (HSCs), ASCs can be enriched based on cell surface markers using fluorescence-activated cell sorting. Even within a specific subset of ASCs sorted by a cell surface receptor, heterogeneity persists. While stem cell populations are known to be heterogeneous, the functional consequences of this heterogeneity have not been fully elucidated. The problem of undefined heterogeneity within stem cells must be overcome before they can be used effectively for therapeutic applications. The first step to understand this heterogeneity is to elucidate the gene expression profiles of these complex cells which can be done using single-cell microfluidic technology (Fig. 13.9). This permits precise mixing of nanoliter quantities of quantitative PCR reagents to perform single-cell transcriptional analysis across single cells.
Methods of ASC delivery
Despite accumulating animal research and intriguing case reports, much remains unknown regarding the optimum mode of ASC delivery. A significant and growing number of studies have examined the intravenous (IV) administration of ASCs. Such studies have investigated the natural distribution of ASCs following IV injection. Interestingly, most organs show uptake of ASCs after administration, including bone and bone marrow tissues, and at least some studies show long-term persistence within the host without oncogenic transformation.71–73 These studies were geared toward disparate interests, but all were rooted in the regenerative capabilities of ASCs. For example, studies have examined IV injection of ASCs for repair of the liver,72 heart,74 endothelium,75 and even olfactory epithelium.76 An emerging interest as well is in the IV administration of ASCs for autoimmune and inflammatory disorders, such as experimental colitis and abdominal sepsis,77–79 muscular dystrophy,80 experimental arthritis,78 and encephalomyelitis.81 Two provocative case studies have also been published in humans showing beneficial outcomes of IV-delivered ASCs: the first in rheumatoid arthritis, and the second in chronic autoimmune thrombocytopenia.82,83 Thus, the use of IV ASCs may be a safe and beneficial future therapeutic modality.
In vitro: protocols on differentiation
Osteogenic differentiation (Fig. 13.10)
For osteogenic differentiation, cells should be plated in a six-well plate at a density of 100 000 cells per well, in 12-well plates at a density of 50 000 cells per well, or in a 24-well plate at 25 000 cells per well. After attachment, ASCs are treated with osteogenic differentiation medium (ODM) containing Dulbecco’s modified eagle medium (DMEM), 10% FBS, 100 µg/mL ascorbic acid, 10 mM β-glycerophosphate, and 100 IU/mL penicillin/streptomycin. This differentiation media can be enhanced to stimulate increased osteogenesis with the supplementiation of several cytokines, such as insulin-like growth factor, platelet-derived growth factor (PDGF), or BMP-2.84,85 ODM should be replenished every 3 days.
Early and late osteogenic differentiation have been defined at different time points which differ across species. Whereas mASCs undergo early osteogenesis by day 7 and late osteogenesis by day 14, hASCs undergo much more robust osteogenesis and should be analyzed at day 3 for early differentiation and day 7 for late differentiation. To assess osteogenic differentiation, RNA can be analyzed from ASCs. Specific gene markers of osteogenic differentiation include osteocalcin (OCN), osteopontin (OPN), runt-related protein-2 (RUNX-2), collagen Ia (COL1A), and alkaline phosphatase (ALP). ALP staining and quantification can also be performed on day 3 of differentiation to assess for early ostegenic activity (Fig. 13.11). Subsequently, late osteogenic activity can be assessed at day 7 of differentiation by alizarin red staining, which detects extracellular mineralization.
Adipogenic differentiation (Fig. 13.12)
To drive ASCs to adipose tissue, cells are seeded in a similar density as described for osteogenic differentiation. Adipogenic differentiation medium contains 10 µg/mL insulin, 1 µM dexamethasone, 0.5 mM methylxanthine, and 200 µM indomethacin. At 3 days, medium should be replaced with 10 µg/mL insulin. Differentiation can be assessed by oil red O staining and quantification or gene analysis. Specific genes expressed by adipose tissue during differentiation that can be analyzed include GCP1, lipoprotein lipase (LPL), and peroxisome proliferation-activated receptor γ (PPARγ: Fig. 13.13).
In vivo model
Nude athymic mouse 4-mm calvarial defect
To translate in vitro findings to the clinical realm, robust in vivo data must be obtained to demonstrate the osteogenic capacity of ASCs. In vivo models should include large immunocompetent animals such as sheep86 and dogs,87,88 as well as smaller immunocompromised animals such as rabbits,89 rats,90 or mice.28 Though several models exist to determine in vivo osteogenic healing, we believe the nude mouse model offers a reliable, easily replicated, and easily followed defect. Immunocompromised animals such as nude athymic mice allow scientists to assess the effect of ASCs on an area while decreasing the innate immune response to xenotransplanted cells which can confound results. Mice are readily available in large quantities and can be studied by most small-animal imaging technologies such as microcomputed tomography (microCT), micropositron emission tomography (micro-PET), and bioluminescent imaging. Mice, however, heal wounds rapidly and effectively and thus scientists must demonstrate that any result is superior to the animal’s baseline healing capacity. One way to demonstrate an effect is to create a defect so large that it overwhelms the innate ability to heal the wound. This large defect, that does not heal when followed over time, is referred to as “critical-sized.” One such defect we have found to serve as an in vivo model is a 4-mm defect in the parietal bone of a nude athymic mouse.69
Nonhealing, critical-sized (4-mm) calvarial defects can be created in the right parietal bone of adult (60-day-old) male CD-1 nude mice using a high-speed dental drill. After cleaning the surgical site with Betadine, an incision is made just off the sagittal midline to expose the right parietal bone. The pericranium will be removed using a sterile cotton swab. Using diamond-coated trephine bits and saline irrigation, unilateral full-thickness critical-size calvarial defects are created in the nonsuture-associated right parietal bone. Importantly, the dura mater should be left undisturbed (Fig. 13.14). In preparation for implantation, scaffolds can be seeded with hASCs 24 hours prior to implantation. For scaffold creation, apatite-coated PLGA scaffolds were fabricated from 85/15 poly(lactic-co-glycolic acid) by solvent casting and a particulate leaching process. Briefly, PLGA/chloroform solutions were mixed with 200−300 µm diameter sucrose to obtain 92% porosity (volume fraction), and compressed into thin sheets in a Teflon mold. After freeze-drying overnight, scaffolds were immersed in ddH2O to dissolve the sucrose, and gently removed from the Teflon plate for disinfection and drying.
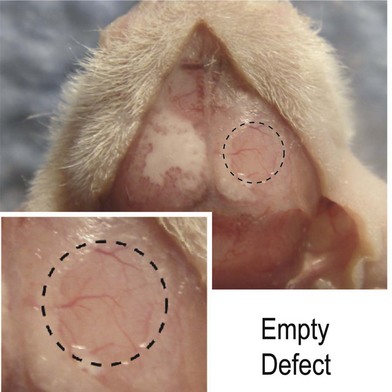
Fig. 13.14 A 4-mm nonhealing critical-sized defect in the right parietal bone of a CD-1 nude athymic mouse.
We found 150 000 cells/scaffold to be optimal in our laboratory.70 The hASCs to be seeded on to each scaffold are suspended in 25 µL of growth media, and placed on to the scaffold for 30 minutes. The scaffold will subsequently be submerged in 100 µL of growth media for 12 hours of incubation. Before implantation, cell-seeded scaffolds should be rinsed in sterile PBS to prevent transfer of medium-derived growth factors.
Clinical correlates
Recent case studies have focused on the use of hASCs to replace bone loss.91 In case reports, defects of the calvaria,92 maxilla,93 and mandible94 have been either healed or enabled to heal faster with the use of hASCs. Such reconstructions eliminate the need for alloplastic materials, and thus reduce the risk of infection, breakdown, or rejection. Despite accumulating translational research, there is a paucity of data defining the mechanisms through which hASCs influence an osseous defect. Do hASCs directly form bone to heal a skeletal defect? In opposition, do engrafted hASCs function as efficient factories to produce potent pro-osteogenic cytokines? In the case of our calvarial defect model, careful examination of calvarial defects engrafted with ASCs yields some profitable insights into the potential derivation of healing. For example, bone is often observed to mineralize from the edges of a cranial defect inwards, which suggests that the host calvarium may contribute to the bony regenerate. Concurrently, small, isolated islands of bone are often observed early postoperatively, which suggests either contribution from engrafted ASCs or alternatively from host dura mater (another source of significant ostogenic progenitors).95–97 Finally, uniform and thorough healing of hASC-engrafted defect suggests primary osseous healing derived from the engrafted donor cells themselves. It is likely that all three cell types (donor ASCs, host calvarial osteoblasts, and host dura mater) contribute to bony healing.
Future direction of tissue engineering using ASCs
A significant gap exists between the current knowledge regarding ASC biology and their future translation to clinical use. First, the safety of hASCs must be determined. Oncogenic transformation must be inhibited, such that tumors of mesodermal origin are not produced after cell engraftment.73 We believe that major breakthroughs in aesthetic and reconstructive surgery using autologous tissue will not come by incremental improvements to technical aspects such as liposuction cannulas or injection syringes. Instead, novel cell-based strategies must be explored to identify and isolate specific fat precursors from hASCs and to coordinate the physiologic induction of adipose tissue differentiation in a biomimetic environment in vivo (Fig. 13.15). hASC-seeded biomaterial constructs may provide a new direction for autologous fat transfer through the assembly of natural three-dimensional adipose, cartilage, or bone tissues.98 Our ultimate translational goal is, during the course of a single operative procedure, to harvest subcutaneous adipose tissue, isolate ASC, and implant these cells on an osteoconductive and/or osteoinductive scaffold into the skeletal defect without leaving the operating room (Fig. 13.16).
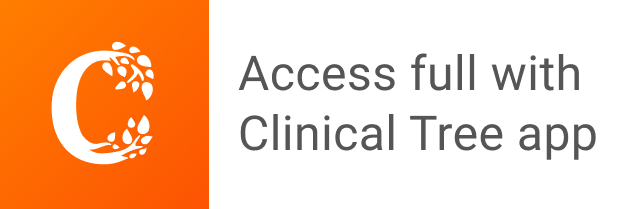