7 Skeletal reconstruction
Synopsis
The reconstruction of defects of the appendicular and axial skeletal has been an evolving clinical endeavor that has mirrored our understanding of the basic science of bone biology.
This chapter serves as an overview of the basic tenets of bone biology, methods of skeletal reconstruction, and a discussion of the most common skeletal defects and how the reconstructive surgeon can reasonably approach them.
Perhaps no other clinical tool has had as much of an impact on the ability to reconstruct skeletal defects as the microsurgical transfer of bone. Thus, no chapter on skeletal reconstruction would be complete without a review of the microsurgical principles of bony reconstruction.
The reader should understand how to approach skeletal defects and the algorithm for their reconstruction upon reading this chapter.
Historical perspective
The quest for a means to reconstruct skeletal defects of the axial and appendicular skeleton has been an ongoing process. Much of the early work on skeletal reconstruction was performed in the craniofacial skeleton. The history of bone grafting dates to the 15th century when Van Meerken transferred a canine bone graft to a calvarial defect. There followed an ongoing debate of which constituents of bone must be transferred for osteogenesis to be observed. Barth observed that, after bone grafting, the graft itself did not survive, but that ultimately grafting worked via replacement of the dead bone by live bone growing into it by creeping substitution.1 An early matter of debate regarding autogenous bone grafting was how much of the graft ultimately survived, and which constituents of the graft were needed for successful autogenous bone grafting. Phemister demonstrated that diffusion of oxygen and nutrients from the underlying recipient bed was likely to result in survival of some of the osteogenic cells on the surface of the bone graft.2 Gallie and Robertson3 noted improved osteogenesis with the use of cancellous bone rather than with cortical bone. This is the result of improved diffusion of nutrients through the cancellous bone rather than through dense cortical bone and the higher content of osteoprogenitor cells in cancellous bone as opposed to cortical bone. In fact, the fate of the cellular content of cortical bone grafts was elucidated in the 1950s and 1960s when it was demonstrated that bone cells within the cortical graft only survived if they were within 300 µm of the surface of cortical bone in contact with a healthy wound bed.4,5
Basic science
Inert bone grafts, including those that are xenogenic, have been shown to be successful through osteoinduction. There are no living cells in devitalized bone grafts, and growth of bone within the implant is dependent on creeping substitution of the living bone that is in contact with the implant via osteoinduction. These inert bone grafts maintain the ability to provoke a morphogenic response and maintain the potential for bone formation. Bone morphogenetic protein (BMP) is responsible for this differentiation and its potential was first demonstrated by Urist in the 1950s and confirmed by several additional authors.6–8 It is this morphogenetic property of xenografts that allowed them to retain the potential for bone formation. Unfortunately, the immune response elucidated by xenografts limits their practical use by preventing their incorporation or revascularization. Urist’s work did confirm the capacity of devitalized bone matrix to induce the formation of bone in heterotopic sites. Ultimately, the process of bone grafting involves osteoinduction, osteoconduction, and osteogenetic potential, which is in part modulated through the BMPs. Osteoinduction is the signaling of the graft to stimulate the formation of new bone, osteoconduction is the underlying framework over which new bone will grow, and osteogenesis is the ability of the bone graft itself to form new bone from its cellular elements. Autologous bone graft has the ability to perform all three and therefore remains the standard to which all others are compared.9
Allografts are, by definition, allogeneic to the recipient. Allografts are functionally strong, but their incorporation remains questionable over their lifespan and they have been associated with relatively high complication rates. These complications include nonunion, fracture, and infection, all of which necessitate their removal.10–12 There are methods to combine the initial structural integrity of the allograft with a vascularized autograft to take advantage of both methods.13 This will be discussed in a subsequent section. The ideal allograft is sterile cortical bone without loss of BMP activity while lowering the antigenicity of the implant. Current methods of preparation attempt to optimize on these principles while leaving enough of the matrix to elucidate the BMP response. Allograft does have the advantage of a potentially unlimited amount of bone for reconstruction and the avoidance of a donor site which has a morbidity associated with it.
Vascularized bone grafts display less resorption than nonvascularized grafts over time, and are able to heal secondary to their maintenance of viable cells within the graft and are capable of healing to the surrounding bone much in the manner of a fracture. These live bone grafts do not heal by the creeping substitution which is observed in inert bone grafts. Much like bone grafting, the use of vascularized bone grafts began in the craniofacial skeleton. Early conventional vascularized flaps were composed of vascularized calvarium that were included in full-thickness scalp flaps.14
The first vascularized bone flap for lower extremity reconstruction was performed in 1905 with the use of a pedicled vascularized fibula for tibial shaft reconstruction. The superior viability of vascularized bone flaps was evident, but with the advent of the operating microscope and microvascular surgery decades away, the routine use of vascularized bone flaps was not possible. In the 1970s there was an explosion of microsurgery techniques and vascularized free bone flaps, many of which took advantage of incorporating a soft-tissue envelope with the underlying bone to form osteocutaneous and osteomyocutaneous flaps (Fig. 7.1). Vascularized bone has the distinct advantage of being alive and able to grow as well as hypertrophy in response to stress, load-bearing, vascularity, and additional environmental signals. The concept of a vascularized bone graft undergoing hypertrophy by environmental stress is exemplified by a free fibula replacing an intercalary segment of a load-bearing bone (Figs 7.2 and 7.3).
The vascular supply to the bone dictates the nature of flap harvest and how best to achieve the most viable bone stock for transfer based on its anatomic vascular pedicle. The blood supply to long bones is derived from multiple sources which have a direct impact on the type of flap that may be transferred. The nutrient artery supplies the marrow cavity and the inner cortex, the periosteal vessels supply the outer cortex of the diaphysis, and the metaphyseal and epiphyseal vessels traverse the cortex and have an anastomotic arcade with the nutrient artery.15 Thus, a long-bone free flap based on the periosteal vessels may have necrosis of the central portion of the graft. Conversely, the nurtrient artery supplies the majority of the vessels to the long bone. The outer cortex may become necrotic if the flap is based solely on the nutrient artery. This concept is well illustrated by a free fibular transfer based on its nutrient vessel to maintain the viability of the graft.
The blood supply to the membranous bones is distinct from the long bones. The typical pedicle to a free flap of a membranous bone is a periosteal pedicle with good filling of the nutrient vessel canals. An example of this type of flap is the free iliac crest flap which is based on a periosteal pedicle derived from the superficial circumflex iliac artery and was first described by Taylor.16 The source of the ideal vascularized bone graft will depend upon the available donor sites, the anatomic location of the skeletal defect in need of reconstruction, and the potential of the patient to heal both the recipient and donor site. The ideal reconstruction is one in which the vascularized graft integrates well into the recipient site with rapid bony consolidation and provides structural stability to the skeletal framework.
Diagnosis/patient presentation
It is the reconstructive surgeon’s responsibility to apply these basic science principles to the specific clinical situation that is encountered in treating patients with skeletal defects. Injuries to the appendicular and axial skeleton are commonly encountered at most major medical centers. Both plastic and orthopedic surgeons are called upon to treat these challenging patients on a routine basis. These patients are best served with the “orthoplastic” approach in a multidisciplinary setting.17,18 The evolution of the orthoplastic approach to limb salvage requires the participation of many different disciplines. The orthopedic and plastic surgeons must be supported within the multidisciplinary orthoplastic approach by prosthetists, physical therapists, vascular surgeons, infectious disease physicians, musculoskeletal radiologists, and a nursing staff well versed in patients undergoing extremity and skeletal reconstruction and convalescence.
One of the key concerns in reconstruction of the appendicular skeleton is the blood supply to the bone graft. If a cancellous or cortical cancellous bone graft is planned, then it is the blood supply of the underlying wound bed that will be responsible for supplying the necessary blood supply to the graft. If there is poor blood supply to the wound bed, the likelihood of success of a nonvascularized bone graft is poor despite a meticulous surgical approach. There is occasionally the clinical situation of a poorly vascularized wound bed for grafting that is secondary to a reversible or treatable flow-limiting lesion. In patients with an abnormal vascular exam, angiography is indicated to discern the blood supply and to determine if there is a reversible flow-limiting lesion that can improve perfusion to the proposed site of grafting. In patients in whom a vascularized bone graft is planned there needs to be adequate inflow and outflow to achieve success. In patients with an abnormal vascular exam with weakly palpable or nonpalpable pulses, it is the authors’ preference to obtain a formal arteriogram to aid in preoperative planning. It has been convention to obtain an invasive arteriogram to delineate the vascular anatomy.19 However, invasive arteriograms are not a morbid-free procedure and computed angiography has become widely available. CT angiography has the advantage of assessing the venous system and surrounding soft tissues as well as the skeletal defect in one study.20 Patients with known vascular disease and limited inflow with soft-tissue defects can be successfully reconstructed with free tissue transfer with acceptable flap success rates if patient selection is appropriate.21,22
One of the persistent questions in the treatment of patients with skeletal defects, particularly in the acute traumatic setting, is discerning who should be offered salvage versus amputation. Much of the data regarding the utility of limb salvage has been derived from the Lower Extremity Assessment Project. Functional outcomes of amputation versus limb salvage for limb-threatening injuries have been extensively studied.23–32 Outcomes between those patients undergoing reconstruction and those receiving amputation are equivalent. Those patients who elect to undergo reconstruction for traumatic skeletal defects will require an increased number of operative interventions compared to those patients undergoing amputations, but if patients are carefully selected they have the potential to have a functional outcome. Obviously, the overwhelming majority of patients desire to keep the injured extremity if at all possible.24,33 Ultimately, the decision to proceed with complex soft-tissue reconstruction with or without skeletal reconstruction of associated defects must be individualized to the patient and his or her potential for recovery.
Treatment and surgical technique
Bone grafting
Traditionally, corticocancellous bone grafts had been used for bony defects that are 5 cm or less in length. Conventional bone grafts require a well-vascularized bed for bone grafting that is free of underlying infection and has adequate soft-tissue coverage. These main tenets of bone grafting were put forth by Kazanjian in the 1950s34 and still hold true today. These principles remain: the recipient site must have adequate blood supply to ensure the survival of the graft, bone-to-bone contact must be established to facilitate creeping substitution, there should be no motion at the fracture site through the use of rigid fixation, and the wound bed must be free of infection. For defects that are greater than 5–6 cm, graft resorption typically prevents complete healing.35,36 However, Masquelet has described a technique of inducing bioactive membrane that has extended the diaphyseal defect for which cancellous bone grafting may prove to be successful.37
The Masquelet technique has been used with success for intercalary defects greater than 5 cm. This technique involves creating an induced membrane to reconstruct large defects with nonvascularized autograft.37,38 The principles of reconstruction with the Masquelet technique begin with the basic tenets of wound preparation: radical debridement of devitalized tissue and delineation of the intercalary defect. If there is a concomitant soft-tissue defect, flap reconstruction to reconstitute the soft-tissue envelope is undertaken to provide for definitive soft-tissue reconstruction. A polymethyl methacrylate spacer is then placed into the defect. The second stage is then undertaken 6–8 weeks later when the spacer is removed and the membrane that is induced by the spacer is left in place. This cavity is then packed with cancellous bone graft derived from the iliac crest. The induced membrane is then closed over the autograft, resulting in a contained system. The induced membrane has been shown to have biologic properties, including a rich vascular network, a synovial-like epithelial lining, and to be biologically active, secreting growth factors such as vascular endothelial growth factor and transforming growth factor beta-1. Additionally, extracts from the membrane stimulated bone marrow cell proliferation and differentiation to osteoblastic cell lines.39 This technique has proven to be powerful in a small series, with the authors able to achieve union of long-bone segmental defects ranging from 5 to 24 cm.38
Vascularized bone transfer
Intercalary defects that are larger than 5 cm typically required free vascularized bone reconstruction. Vascularized bone grafts have a significant advantage over their nonvascularized counterparts.40 Additionally, there may be an additional soft-tissue defect associated with the underlying bone loss and an osteocutaneous free flap may be used to provide simultaneous skeletal as well as soft-tissue reconstruction. Vascularized bone grafts for use as a free flap and microsurgical reconstruction of the skeleton have become commonplace in most major medical centers and can be done with a very high patency rate of the graft. Additional factors to consider when choosing a free vascularized bone graft is the available pedicle length that is characteristic of the flap, the available bone stock of the flap in terms of its length and thickness, its osteogenic potential, and ease of harvest at the time of surgery if concomitant orthopedic procedures are to be performed.
Since it was first described in 1975,41 the fibula has become the preferred source for vascularized bone grafts for reconstruction of defects of the axial as well as appendicular skeleton. The fibula is well suited for use as a free vascularized bone graft given its potential for an abundant source of the bone, a long length of vascularized graft, and acceptable donor site morbidity.41 Up to 26 cm of vascularized cortical bone can be harvested from the fibula in the typical adult patient. The fibula is triangular in shape and primarily a cortical bone with a small medullary component. These anatomic characteristics allow it to resist angular and rotational stress and to remodel with graduated weight-bearing in the postoperative period for intercalary defects. The blood supply to the diaphysis of the fibula is based on an endosteal and musculoperiosteal component which are both provided by the peroneal artery and vein. In a small (<1%) number of patients the peroneal artery is the dominant supply to the lower extremity.42 Peroneus magnus may not be evident on preoperative clinical exam, but if it is encountered intraoperatively the fibular harvest should be abandoned so as not to render the foot ischemic. If the patient has an abnormal pulse exam or has a traumatized extremity that suggests possible underlying vascular injury, preoperative imaging is indicated. A skin paddle may be harvested with the fibula to reconstruct associated soft-tissue defects concomitantly. The skin paddle is supplied by perforators that exit through the posterior crural septum and are found in greatest concentration at the proximal and distal fibula (Fig. 7.4).
The epiphysis of the fibular head is supplied by the anterior tibial artery. If epiphyseal growth of the fibula is needed for skeletal reconstruction in the immature skeleton to allow for longitudinal growth, the epiphyseal blood supply needs to be included in the graft so that survival of the epiphysis is insured. It has been shown in studies by Taylor et al.43 and Bonnel et al.44 that the proximal diaphysis is supplied by small, musculoperiosteal branches and there is no need to harvest both pedicles if there is only a small, proximal diaphyseal segment needed for reconstruction. If a significant length of the fibular diaphysis is needed, then both the anterior tibial and peroneal artery must be included with the graft to ensure adequate survival of the segments (Fig. 7.5). Typically, a thin cuff of muscle (1 mm) is usually left attached to the graft in order to preserve the muscular periosteal circulation and the distal 6 cm of the fibula should be preserved in adults in order to maintain ankle stability. If there is a question of ankle stability following harvest of the fibula, a syndesmotic screw should be placed for additional stability of the ankle joint.
Harvest of the fibula is not without its consequences. Large series have demonstrated persistent long-term deficits after free fibula harvest. Patients may have pain, ankle instability, and/or weakness after harvest of the fibula. Up to 11% of patients undergoing harvest of the free fibula may have persistent pain.45 The incidence of motor weakness decreases over time after harvest of the fibula but persists in a subset of patients. A small number of patients will complain of subjective weakness in the follow-up period. However, when the harvested legs are compared to the nonoperated leg with isokinetic testing, strength measurements at the knee and ankle are significantly decreased.46 These defects rarely cause a disability in the patient’s postoperative functioning and should not prohibit the reconstructive surgeon from harvesting the appropriate free vascularized fibular graft for reconstruction.
The iliac crest vascularized bone flap was popularized in the early 1980s. Its vascular supply is based on the deep circumflex iliac artery. It has the advantage of being able to supply a large segment of bone as well as skin. A segment of bone up to 4 × 11 cm can be harvested as a vascularized segment with a skin paddle measuring 8 × 18 cm. The curve of the native ileum limits its usefulness in intercalary defect reconstruction secondary to its curvature. In general, reconstruction of intercalary defects with the free iliac crest flap is limited to 10 cm. If the defects are more than 10 cm, then osteotomies are required to account for the curvature of the graft. Additionally, there is in inherent risk of hernia after harvest of this flap and anesthesia in the distribution of the lateral femoral cutaneous nerve of the thigh. In a large series, 9.7% of patients developed a formal hernia after harvest of the flap. These donor site hernias are challenging to repair secondary to their anatomic location. Persistent pain is a problem in approximately 8% of patients at 1 year after harvest.47
Periosteal and other bone flaps
Periosteum has long been known to have significant osteogenic activity.48,49 The osteogenic properties of the periosteum can be utilized by harvesting a vascularized periosteal flap. Periosteal and osteoperiosteal grafts from the medial femoral condyle were first used as pedicled flaps50 and subsequently as free flaps.51 Recently, the free vascularized medial femoral condyle flap has been shown to have a plentiful and reliable blood supply based on the descending genicular artery52 and has been used to treat recalcitrant nonunions of the appendicular skeleton. The size of the vascularized bone that is available is ideal for smaller nonunions. The vascularized medial femoral condyle flap may be harvested as an osteogenic periosteal, an osteoperiosteal, or a cutaneous osteoperiosteal flap depending on the needs of the reconstruction. Early experience in the use of this flap has been primarily with scaphoid nonunions, but has also proven useful in nonunions of long bones and mandibular defects as well.53–57 This flap is based upon the descending genicular artery, which is a branch of the superficial femoral artery, and on the superomedial genicular artery, which is a medial branch of the popliteal artery. The pedicle is reliable and between 1.5 and 3.5 mm in diameter at its origin. The medial condyle has a rich supply of arterial perforators to facilitate harvest of a vascularized bone graft.52 This flap lends itself well to the harvest of a smaller vascularized bone graft for the treatment of recalcitrant nonunions. Use of this flap for nonunions displayed a 75% success rate for primary union without complication over an average time period of 3.8 months.53 Given the reliability of the pedicle and the rich vascular supply of the medial femoral condyle, this flap has great utility in both the axial and appendicular skeleton.
Distraction osteogenesis and the Ilizarov technique
The Ilizarov technique is a means of generating bony length through distraction osteogenesis as well as the capacity to generate additional soft tissue. The Ilizarov technique was developed in Siberia in the 1950s when Ilizarov observed that bone and soft tissue could be generated through the use of judiciously applied tension.58,59 The Ilizarov technique can be used for reconstruction of defects up to 15 cm and may be combined with free vascularized bone grafts in order to achieve reconstruction.60,61 The Ilizarov method may be used to treat fractures, nonunions, segmental loss of bone, malrotation, and congenital abnormalities. The Ilizarov technique requires the use of an external fixator that is rigid but allows axial motion. The Ilizarov device is a system of rings and thin wires that transfix the extremity at a tension of 60 and 130 kg.62 The Ilizarov technique is incredibly powerful in its ability to adjust for deformity of the extremity and to generate bone and soft tissue as the limb is distracted (approximately 1 mm/day) over small increments. Muscle, nerves, blood vessels, and skin are generated in response to the tension applied by the frame and the components proliferate at the cellular level in response to tension.63,64 In many patients, particularly those patients with Gustilo IIIB tibia fractures, there may be associated soft-tissue loss with an underlying bony defect. The Ilizarov method may be combined with free tissue transfer of soft tissue alone, or with a free vascularized bone flap that may subsequently be distracted (Fig. 7.6). The utility of this combined technique was first elucidated by Fiebel et al. in 1994 and subsequently expanded upon by numerous others.65–67
The use of the Ilizarov frame in relation to soft-tissue reconstruction may be thought of in the framework as elucidated by Hollenbeck and colleagues.68 In type I patients the Ilizarov frame may function as an external fixator for stabilization. In this case, there is adequate bone for fracture healing and the need for soft tissue can be managed with a pedicled or free tissue transfer. In type II patients the Ilizarov frame may be placed after free tissue transfer for ongoing correction of a potential nonunion or malunion. In type III patients the Ilizarov frame is placed in conjunction with a free flap and immediate bone shortening and corticotomy. The patient subsequently undergoes distraction in order to compensate for bone loss and limb length discrepancies. In type IV patients, the Ilizarov frame is used as a method of fixation for a free vascularized bone graft when the defect meets the criteria for the need of free vascularized bone transfer. This classification scheme is a useful construct to stratify the treatment of patients requiring the Ilizarov method and soft-tissue reconstruction.
The placement of the Ilizarov frame and soft-tissue reconstruction may be staged. In those patients who have a hostile or inadequate wound bed with residual infection, an antibiotic spacer may be placed in the skeletal defect at the time of debridement and soft-tissue reconstruction. Initial provisional fixation is provided by an external fixator followed by placement of an antibiotic-impregnated methylmethacrylate spacer, followed by definitive soft-tissue coverage. This allows a stable soft-tissue envelope at the time of placement of the Ilizarov frame.69
There have been recent advances in the construct of the frame itself. The Taylor spatial frame has shown promise in the treatment of traumatic injuries with acute bone loss as well as tibial nonunions with associated bone defects.70–73 The spatial frame differs from the standard Ilizarov construct in that it is a thin wire frame with virtual hinges and computer software support to facilitate fine corrections of bone length, angulation, and rotation. The spatial frame allows the reconstructive surgeon to have control over multiple variables through the utilization of a hexapod construct, and sophisticated computer algorithms to correct underlying deformities without frame modification, as is the case with a standard Ilizarov frame. Additionally, the spatial frame allows for potential early weight-bearing and range of motion, which may lead to improved rates of union.
Allograft reconstruction
Reconstruction of skeletal defect greater than 6 cm in length will require allograft or vascularized bone reconstruction. The reconstruction of large bony defects can be problematic and has limited surgical options. Allografts provide for reconstruction with a readily available product that can be cut to the desired size and lack the morbidity of a donor site that is required when harvesting a vascularized autograft. Allografts are typically cryopreserved grafts that are cataloged and then selected based on their specific anatomic characteristics and the reconstructive needs.74 However, these are nonvascularized grafts and ultimately lack any osteogenic potential. The healing of these nonvascularized grafts is slow, and their incorporation is never complete.
Most studies of allografts conclude that the inner portion of the allograft remains a mechanical graft without cellularity and replacement with living tissue with the outer 2–3 mm is gradual75–77 Allografts may be intercalary or osteoarticular. The union times of the allograft vary according to the anatomic position of the allograft, with osteoarticular grafts typically healing in less time than intercalary grafts. Union times for allograft can be as long as 23 months in the intercalary position, with union in the osteoarticular position taking as long as 12 months.75,76,78,79 Many of the patients in need of a large allograft are patients who have an underlying bony malignancy that will require chemotherapy, which may further delay healing and union of the allograft. Despite their widespread use and availability, the avascular nature of these grafts predisposes them to complications, including infections and fracture and the need for revisions in the future (Fig. 7.7).
The use and subsequent success of allografts remain dependent on the surrounding soft tissues. If there is an insufficient soft-tissue envelope, or if the surrounding soft tissue has been previously radiated, one can expect a higher complication rate which includes allograft-related infections, fractures, and nonunions.12,80–84 When one looks at large series of long-bone reconstruction with allografts, there is a failure rate of 14% for intercalary grafts, and a wide-ranging complication rate,85 including a nonunion rate up to 30% and failure rate of 37%.86 The majority of these failures occur within the first 3–4 years following reconstruction, with return to function being much better for allografts in the intercalary position than when combined with a prosthesis or when used in an allograft arthrodesis.74 In fact, when one examines the literature regarding the use of allograft reconstruction, intercalary allograft reconstruction fares much better than any other.12,83,87–89 This fact has bearing on the current discussion as most reconstructive surgeons will be primarily involved in reconstruction of intercalary defects, with most osteoarticular or reconstructions combined with a prosthesis being performed by an orthopedic surgeon.
The Capanna technique takes advantage of both allograft and free vascularized bone reconstruction of the femoral shaft.13,90–94 The intercalary allograft provides initial stability and mechanical strength, while the free vascularized bone allows integration, the capacity for bony remodeling, and long-term viability of the construct and bony union. The technique involves cutting the cortical allograft to the appropriate size of the skeletal defects. The medullary portion of the allograft is then enlarged to facilitate placement of the free fibular bone graft within the medullary canal. Typically, the ends of the free fibula are left longer than the allograft so that they will have overlap with the proximal and distal ends of the bony segment. A canal or trough is cut within the allograft to provide for the vascular pedicle and to facilitate microvascular anastomoses. This method can be used to reconstruct defects of the femur, tibia, and humerus. It may be used in the immediate setting after tumor resection, or in a delayed setting for nonunion of a previously placed allograft. For patients undergoing resection for sarcoma and immediate reconstruction, the allograft will provide mechanical stability and allow for earlier ambulation without the requisite delay for hypertrophy of the free fibular graft. Additionally, patients undergoing resection for sarcoma may have delayed healing secondary to the need for adjuvant chemotherapy which would otherwise delay healing of the free fibular graft. Using the Campanna technique, recent studies have displayed a mean time for bony union of 8.6 and 9 months respectively.13,93
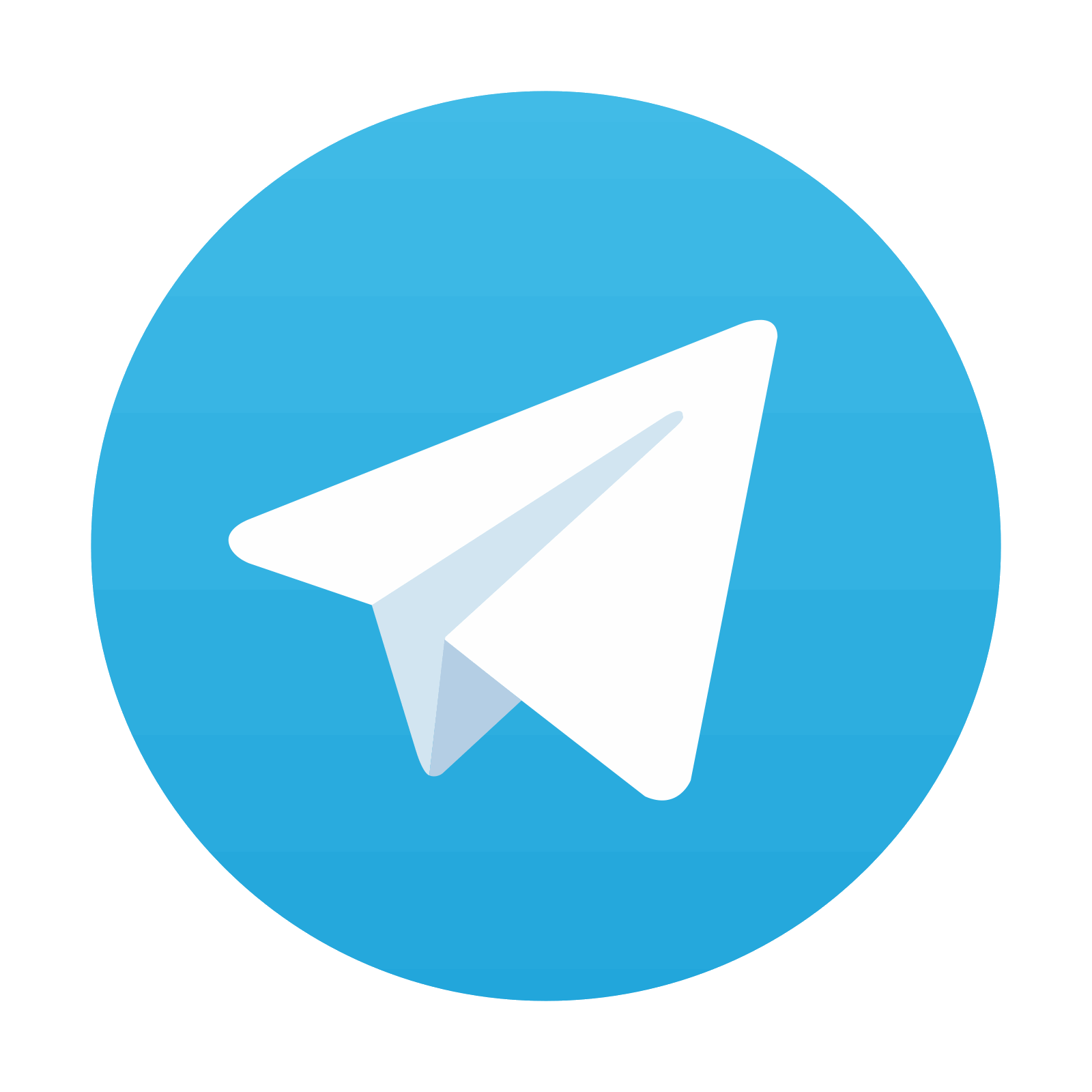
Stay updated, free articles. Join our Telegram channel
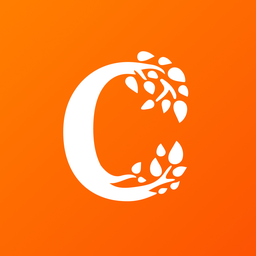
Full access? Get Clinical Tree
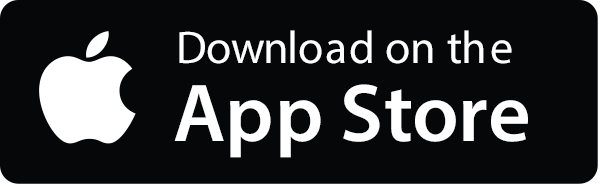
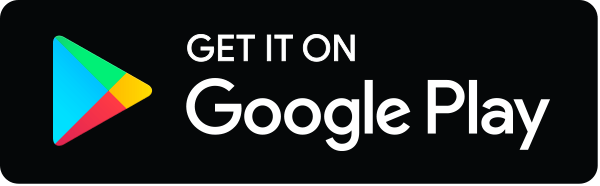