Fig. 15.1
Vascularized composite allotransplantation achieves high-quality restoration of function and form following complex injuries to specialized anatomic subunits
However, despite these considerable benefits and the positive clinical outcomes to date, VCA transplantation is not, in contrast to solid organ transplantation , an acutely life-preserving procedure. Therefore, the risk-to-benefit ratio of the surgery, the necessary lifelong immunosuppressive therapy, and the sequelae of immunologic rejection, whether acute or chronic, must be carefully considered. Indeed, the ethical aspects of VCA transplantation, particularly with regards to face transplants , remain a topic of considerable debate [11–13]. While various immunosuppression minimization approaches have shown considerable promise, and are undoubtedly closer to clinical application for VCA if not already in clinical trial [14], we believe that transplant tolerance, defined as a state of specific unresponsiveness to donor antigen, permitting long-term rejection-free acceptance of transplanted tissues without immunosuppression, represents the ultimate goal of research in this field. The potential significance of transplant tolerance for VCA was recognized prior to the first face transplant, by the 2004 working party of the Royal College of Surgeons who stated that “clearly, if it did prove feasible to induce transplant tolerance clinically, this would overcome all the immunological disadvantages of facial transplantation. Indeed, many of the ethical objections to proceeding with clinical evaluation of the procedure would be eliminated” [12]. While clinical evaluation of face transplantation has clearly proceeded, and grafts have been designed to restore a range of injuries from partial loss of the skin and muscles of facial expression [15] to complex three-dimensional craniomaxillofacial defects [16–18] with impressive results, recipients of face transplants , and other VCAs, have experienced a burden of immunosuppressive side effects comparable to that observed in solid organ transplantation, including infection, metabolic and renal impairment, and malignancy [3]. Given the necessity for lifelong immunosuppression to prevent VCA rejection, it can be expected that the impact of these side effects will accrue over time, further highlighting the benefit of a tolerance protocol when one considers that the traumatic extremity and craniofacial injury suitable for reconstructive transplantation are predominantly experienced by a relatively young cohort of patients.
While numerous VCAs have now been performed, the incidence and presentation of chronic rejection in these grafts have not yet become clear. To date, allograft vasculopathy has been reported in one knee transplant [19] and one hand, which presented with progressive ischemia necessitating amputation 9 months post transplant, with widespread intimal hyperplasia of donor arteries demonstrated on histology [20] . While these cases may be attributed to chronic rejection, nonimmune factors may also have contributed, particularly in the hand where procurement of a long segment of isolated donor brachial artery and over use of the hand post transplant were described [20]. In contrast to the small number of confirmed cases of chronic rejection to date, the incidence of acute rejection episodes has been reported to exceed 85 % at 1 year [3], and this has been associated with an increased risk of chronic rejection in animal models of VCA [21]. The apparently low incidence of chronic rejection in VCAs may include some degree of artifact due to the relatively small number of cases available for analysis, but none the less stands in contrast to kidney transplantation, for example, where 10-year survival for living donor transplants is 59 % and only 43 % for cadaveric grafts [22]. Chronic vasculopathy has been associated with humoral immunity and the development of donor-specific antibody in various experimental models of organ transplantation [23], and unfortunately has proved resistant to conventional immunosuppression , despite the considerable improvements observed in control of acute rejection over the past 20 years. Until such time as multicenter cohorts of VCA recipients are available for long-term follow-up and analysis, it is likely that experience in organ transplantation will continue to offer important insights for development of VCA protocols, and further investigation of chronic rejection in the context of VCA would be welcome. Induction of VCA tolerance not only avoids the risks associated with long-term treatment with conventional immunosuppressive regimens but also holds the potential to prevent chronic rejection.
Tolerance and Mixed Chimerism
Tolerance of transplanted organs or tissues, including skin grafts, may be achieved in small animal models by numerous protocols; however, nearly all have failed in large animal studies, which remain an important step in the translation from bench to bedside [24]. Hematopoietic mixed chimerism , defined as the coexistence of hematopoietic cells of donor and recipient origin within an individual has been successfully applied for induction of transplant tolerance in small animals [25], in large animal models [26], and recently in clinical trials in renal transplantation [27–29].
The initial association of hematopoietic mixed chimerism with immune tolerance stemmed from an observation in naturally occurring bovine chimeras (termed free-martin cattle) almost 70 years ago [30]. Medawar and colleagues confirmed this observation in a mouse model by intrauterine injection of fetuses with a preparation of cells from a mismatched adult donor, with subsequent skin grafts from the donor strain accepted indefinitely following birth and maturation [31]. Later experiments by Ildstad and Sachs demonstrated the induction of chimerism and tolerance of transplanted skin grafts in adult mice reconstituted with a mixture of donor and recipient bone marrow following myeloablative conditioning [32]. Ablation of the recipient immune system and hematopoiesis, combined with depletion of mature T cells from the donor bone marrow prior to transplant, permitted engraftment of donor hematopoietic cells while avoiding graft versus host disease (GvHD) and permitted development of a new immune system tolerant of donor and recipient antigens. However, these early protocols consisting of lethal total body irradiation (TBI) and chemotherapeutic agents would be unacceptable for use in patients for the sole purpose of inducing VCA tolerance. Furthermore, rodents have also demonstrated considerable resistance to GvHD following hematopoietic stem cell (HSC) transplantation across major histocompatibility complex (MHC) barriers in comparison to large animal models and humans. Paired with a considerable incidence of engraftment failure when the donor hematopoietic cell graft is T-cell-depleted prior to transplant to reduce the risk of GvHD [33–36], the use of myeloablative protocols becomes unjustifiable.
Thus, subsequent studies focused on development of progressively less toxic, nonmyeloablative conditioning regimens based on T-cell-depleting antibodies, low-dose total body, or targeted thymic irradiation and/or costimulatory blockade [37]. Protocols of this sort have resulted in engraftment of donor HSCs and stable multilineage mixed chimerism and induction of donor-specific unresponsiveness with considerably reduced morbidity and mortality. Indeed, this approach was successfully extended to induction of chimerism and organ transplant tolerance [38–40], and more recently VCA tolerance [41, 42] across MHC barriers in the Massachusetts General Hospital (MGH) miniature swine model.
Mixed chimerism has also been successfully applied for the induction of kidney allograft tolerance across MHC barriers in nonhuman primates [26], and in both human leukocyte antigen (HLA)-matched and HLA-mismatched settings in humans [27–29, 43]. Interestingly, both stable [28] and transient [27] mixed chimerism have been demonstrated to contribute to tolerance of renal allografts in clinical trials. The successful induction of kidney tolerance by transient mixed chimerism is consistent with previous data in both small and large animal models achieving induction of renal allograft tolerance by very mild regimens, in which the kidney has been demonstrated to contribute to its own tolerance [44]. This suggests that in the transient chimerism model in nonhuman primates and clinical protocols, the transplanted kidneys may be contributing to the maintenance of tolerance following loss of mixed chimerism, and the mechanisms may or may not be similar for VCA tolerance. The recent report of a protocol achieving engraftment of HSCs, durable high-level chimerism, and acceptance of kidney transplants without long-term immunosuppression from HLA-mismatched donors [28] is an interesting development while may be of significance for more immunogenic transplants, including VCAs, which lack the tolerogenic capacity of renal or liver grafts. All in all, there is currently no one protocol which will reliably and safely induce stable multilineage mixed chimerism across MHC barriers in humans without GvHD, and further work in this area is undoubtedly required.
Mechanisms of Tolerance in Mixed Chimerism
Definitions of immune tolerance vary , but throughout this discussion we will define tolerance as a state of specific unresponsiveness to donor antigens associated with long-term rejection-free acceptance of transplanted tissues without immunosuppression. Currently, mechanisms for induction and maintenance of immune tolerance are considered in three main groups: deletion, anergy, and regulation/suppression. These mechanisms may be further classified as occurring centrally, referring to intrathymic events such as the clonal deletion of alloreactive thymocytes, or generation of T regulatory cells (Tregs) , or peripherally within either secondary lymphoid tissues or at the graft site itself. Similarly, the relative importance of each mechanism may vary during induction and maintenance phases of tolerance, and in protocols achieving transient or limited, rather than stable, multi-lineage mixed chimerism. We will consider each of these mechanisms and scenarios in turn, with a specific focus on tolerance mediated by mixed chimerism, and its relevance to VCA Fig. 15.2 .
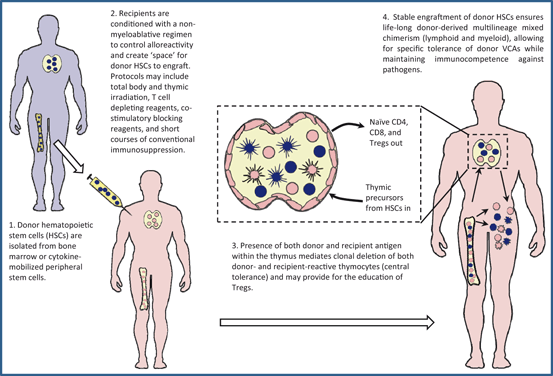
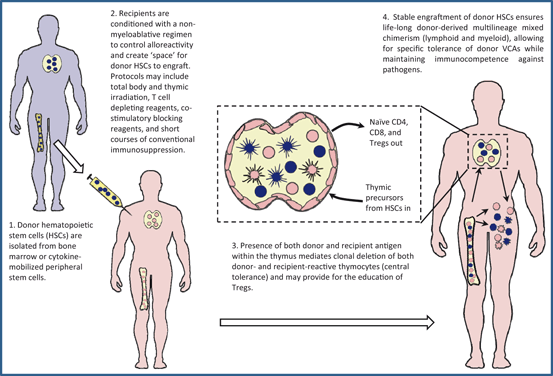
Fig. 15.2
Mechanisms of tolerance in mixed chimerism. VCA vascularized composite allograft, CD cluster of differentiation
Central and Peripheral Mechanisms of Tolerance
The establishment of immune tolerance is a natural process during immune development, which is essential for discrimination of “self” and “nonself,” recognition of pathogens, and avoidance of autoimmunity. If one considers HSC transplantation and the establishment of mixed chimerism as the redefinition of self, it is coherent that the mechanisms by which transplant tolerance is achieved will overlap with those establishing self-tolerance during natural immune system development. Induction of tolerance of donor tissues or organs requires specific unresponsiveness of those immune cells which would otherwise be capable of exerting a destructive effect on the graft. Although cells of the innate immune system, particularly natural killer (NK) cells, have been implicated in some situations [45, 46], allograft rejection is principally mediated by the adaptive immune system. Furthermore, while it has been demonstrated that B cells may be directly tolerized by mechanisms including deletion [47, 48] and anergy [49, 50], evidence for humoral rejection in VCA is lacking [20, 51], and induction of T cell tolerance may be sufficient for tolerance of donor antigens at a functional level [52]. Therefore, we will focus our discussion on the mechanisms of T cell tolerance, as these appear most relevant to VCA.
The thymus is the primary site of T cell development, and also the first step in establishing tolerance at the “population” level. Thus, T cell tolerance established by intrathymic mechanisms is classified as central tolerance. In contrast, peripheral tolerance is established in mature T cells following encounter with antigen in the peripheral tissues. The underlying mechanisms by which both central and peripheral T cell tolerance can be established include deletion, anergy, and regulation. In the context of self-tolerance, peripheral mechanisms were historically viewed as critical in tolerizing T cells, recognizing tissue-specific antigens which may not have been encountered in the thymus during development through interaction with either thymic epithelium or hematopoietic-derived antigen-presenting cells (APCs) [53,54]. Furthermore, it has now been demonstrated that a considerable number of so-called tissue-specific antigens are in fact expressed in the thymus, under the control of the autoimmune regulator (AIRE) transcription factor and can mediate central tolerance of these antigens [55]. Thus, with regard to induction of VCA tolerance, the efficiency of these mechanisms may reflect the challenge of establishing skin tolerance, even in mixed chimeras, as presumably the skin expresses tissue-specific antigens not presented to developing thymocytes during the time of central tolerance induction. Nonetheless, the role of AIRE in mixed chimerism and transplant tolerance has not been established and peripheral mechanisms are likely of considerable importance, particularly in controlling mature alloreactive T cells which survive pre-transplant conditioning.
Clonal Deletion
Clonal deletion is the core mechanism of central tolerance, mediated in the thymus by recognition of autoantigens presented by medullary and cortical thymic epithelial cells and dendritic cells of hematopoietic origin at the cortico-medullary junction [56, 57]. During T cell development, lymphoid progenitors arising in the bone marrow traffic to the thymus and enter the subcapsular region of the cortex where they proliferate and sequentially express cluster of differentiation (CD)2, CD44, and CD25 and undergo rearrangement of the T cell receptor (TCR) beta chain [58]. Successful rearrangement and cell-surface expression of the beta chain with CD3 and pTa, forming the pre-TCR, is followed by expression of CD4 and CD8. These double-positive thymocytes commence alpha chain rearrangement and migrate toward the cortico-medullary junction. Positive selection, mediated by binding of the rearranged TCR to complexes of MHC and self-peptide on cortical epithelial cells with sufficient affinity to provide a survival signal, and ensure cognate T cell/APC interaction in the periphery is essential within 3–4 days to avoid “death by neglect.” But those thymocytes with TCR with high affinity for self-peptide/MHC expressed by hematopoietic-derived dendritic cells at the cortico-medullary junction undergo deletion, also termed negative selection, or clonal diversion to become Tregs [59]. Successfully selected thymocytes differentiate into CD4 and CD8 single-positive populations and migrate to the thymic medulla, expressing high levels of rearranged αβ TCR and addressins to permit homing to secondary lymphoid organs as naïve T cells.
In mixed chimeras, while thymic epithelial cells remain of solely recipient origin, hematopoietic cells of both donor and recipient origin migrate to the thymus and mediate deletion of both donor-reactive and host-reactive T cell precursors . This results in establishment of a peripheral T cell repertoire tolerant of both donor and host, thus simultaneously controlling graft rejection and GvHD [60, 61]. Furthermore, the persistence of recipient-origin APC populations, alongside cells of donor origin, in the periphery of mixed chimeras facilitates appropriate recipient-restricted immune responses and maintenance of immunocompetence. This is in contrast to full chimeras prepared by myeloablative conditioning, in which the MHC disparity between the thymic epithelial cells responsible for positive selection, and the entirely donor-derived APC pool in the periphery against which the recipient-restricted T cells are significantly limited in mounting an effective response [25] .
One of the primary mechanisms by which mixed chimerism maintains long-term, robust tolerance is through the continual presence of donor-derived cells mediating negative selection of newly developing thymocytes. This was demonstrated in a murine model, in which elimination of chimerism using donor-specific anti-MHC antibody resulted in abrogation of tolerance and emergence in the periphery of T cells recognizing donor-presented superantigen. In contrast, if recipient thymectomy was performed prior to elimination of chimerism, donor-specific tolerance was preserved and cells with donor-reactive TCR could not be detected [62]. This result is consistent with a central deletional mechanism of tolerance playing a primary role in maintenance of tolerance in this case, since alternate mechanisms such as anergy and regulation typically required persistence of antigen for maintenance of tolerance.
While central deletion of donor- and recipient-reactive T cells is a highly robust mechanism of tolerance, as cells deleted in the thymus will never be available in the periphery and therefore unable to contribute to pathology, the thymus does not express all antigens expressed by all peripheral tissues. Deletion of mature T cells in the periphery has been described for both CD4 and CD8 T cells in antigen-specific manners [63–65]. Peripheral deletion occurs in a similar fashion to central deletion, through apoptotic cell death, with evidence for both Fas- and Bim- apoptosis pathways operating in a coordinated manner to achieve elimination of chronically self-stimulated T cells in vivo [59] .
Achieving robust tolerance is clearly of significant importance in the development of protocols for clinical application, and the strength of deletional tolerance has been well established, permitting acceptance of highly immunogenic grafts (including skin and small bowel) across stringent histocompatibility barriers in small animal models [66–68]. Given the well-established challenges of inducing tolerance of transplanted skin, and the facility with which mixed chimerism achieves clonal deletion, it is likely that protocols achieving VCA tolerance will be built on a primarily deletional mechanism. However, other mechanisms, principally anergy and regulation, may contribute, particularly in the early post-transplant phase, prior to completion of the deletion process [69] .
Anergy
The classic definition of anergy is a state in which a T cell is rendered unresponsive to an antigen by binding of a peptide-MHC complex (pMHC) in the absence of appropriate costimulatory signals [70–72]. Cellular anergy has been associated with reduced interleukin (IL)-2 production, altered intracellular signaling and tyrosine phosphorylation patterns [73–75]. Recent studies have demonstrated that inhibition of the mammalian target of rapamycin (mTOR) pathway following T cell activation via CD3 and CD28 is sufficient for induction of anergy, and that nutrient-sensing pathways such as activation of the hypoxia and adenosine triphosphate (ATP) deprivation sensor adenosine monophosphate (AMP)-activated protein kinase (AMPK), may play a role in this process [76].
TCR engagement in the context of co-inhibitory molecule ligation such as programmed death 1 (PD-1) and cytotoxic T lymphocyte antigen-4 (CTLA-4) has also been shown to induce an anergic state in T cells. Binding of PD-1 by its ligands PD-L1 and PD-L2 can limit the expansion of autoreactive T cells [77]. Similarly, CTLA-4 binding of CD80/86 can transduce a negative signal that blocks the cell cycle progression [78]. Interestingly, Tregs may promote local hypoxia via expression of CD39 and CD73 which convert ATP to AMP, and AMP to adenosine, respectively, suggesting that the Tregs may in part modulate T cell activity through anergy [79, 80]. Overall, a constellation of genes has been associated with anergy, and interestingly many of which overlap with the expression profiles of cells undergoing peripheral deletion [59, 81] .
Evidence for anergy in mixed chimerism was demonstrated in a murine bone marrow transplantation (BMT) model , using a costimulatory blockade-based induction regimen. Peripheral donor-reactive CD4 T cells were rapidly rendered anergic post transplant, with evidence of complete unresponsiveness to donor at week 1, prior to progressive deletion over a period of weeks [82]. Similarly, the sequential deletion of T cells following induction of anergy has been reported in several other studies [83, 84]. However, induction of anergy does not always precede deletion, as anergic T cells have been shown to survive long term [85,86]) and may in fact exhibit regulatory activity by modulating the activity of other effector T cells [87, 88].
Classically [89], but not universally [90], T cell anergy may be abrogated in vitro by reexposure to cognate antigen in the presence of exogenous IL-2 . There is also evidence for reversal of anergy in vivo both by infection [91] and removal of antigen [92–95]. Clearly, these findings would raise concern regarding the reliability and robustness of any protocol in which anergy was the primary mechanism for maintenance of tolerance over the long term .
Regulation
Specialized cell populations, initially termed “suppressor cells,” capable of attenuating and controlling immune responsiveness in an antigen-specific manner, were identified in the 1970s [96, 97]. Efforts to define the cellular characteristics and molecular mechanisms responsible for suppression achieved limited success until 1990 when it was demonstrated in a rodent model of cardiac allograft transplantation that tolerance could be adoptively transferred by CD4+CD25+ cells [98]. Subsequently, considerable strides have been made in characterizing this cell population, now referred to as Tregs, in both mice and humans [99, 100].
One of the major hurdles in dissecting the specific roles and contribution of Tregs to transplantation tolerance is the heterogeneity of both cell surface marker and transcription factor expression in various model systems and species. While regulatory function has been demonstrated for non-CD4+ cell populations including CD8+ [101–103], CD8+CD28− [104], TCR+CD4−CD8− [105], natural killer T (NKT) [106, 107], and myeloid suppressor cells [108], CD4+ Tregs remain the most fully characterized. The cardinal markers associated with this population of Tregs in humans are considered to be cell-surface expression of CD25 (the alpha subunit of the high affinity IL-2 receptor), constitutive expression of the transcription factor forkhead box P3 (FoxP3) and both cell-surface and cytoplasmic expression of the co-inhibitory receptor cytotoxic T lymphocyte antigen 4 (CTLA-4) [109]. Each of these molecules has been demonstrated to be of functional significance in Tregs, respectively providing survival signals to the Treg (and presumably mediating suppression by IL-2 absorption), promoting transcription of factors essential for Treg development and survival (including CD25 and FOXP3), and outcompeting CD28 for binding of CD80 and CD86 on APCs [110] . Furthermore, Tregs may downregulate expression of CD80/CD86 via a CTLA-4-dependent mechanism, exerting suppressive activity through APC modification [100, 111]. Another area of active research in Tregs that has direct implication for the field of transplantation is the origin of Treg generation. It has been shown that Tregs can arise both during thymic development through intermediate-affinity interactions with antigens expressed in the context of MHC class II on nonhematopoietic thymic epithelial cells in the cortex (nTregs) [112–114] or from naïve CD4 T cell precursors in the periphery (iTregs) [115].
While the principle role of Tregs is maintenance of self-tolerance, and resolving active immune responses to pathogens, they are also the focus of considerable research in the field of transplantation, where they have been demonstrated to play a role in prevention of organ allograft rejection [116–118] and to attenuate GvHD following BMT [53, 119]. Populations of cells enriched for Tregs have also been identified in allograft recipients, both in the peripheral lymphoid tissues [116] and at the graft-site itself [120]. Indeed, FoxP3 + cells have been identified in the skin of accepted hand transplants as much as 6 years post transplant on conventional immunosuppressive protocols [121], and experimentally, in the skin of VCAs transplanted between MHC-matched canines [122].The homing and activation of Tregs are now recognized to mirror that of conventional T cells, and appear to be critical for optimal protection of transplants, including skin grafts, against anti-allo responses [123, 124]. However, as yet the functional significance of Tregs for survival or tolerance of VCAs has not been clearly demonstrated.
The evidence for Treg function in the maintenance of tolerance in mixed chimerism models is sparse [125], but during the induction phase of chimerism, or in models where only low levels of chimerism are achieved, it is conceivable that the contribution of regulatory mechanisms to tolerance may be more significant [126, 127], as in this setting the deletion of donor-reactive T cells appears to be less complete [128] Fig. 15.3.


Fig. 15.3
Tolerance is induced rapidly following hematopoietic stem cell transplantation, with the contribution of nondeletional mechanisms declining over time as clonal deletion progresses
Mixed Chimerism: Transient Versus Stable
In elucidating and determining the contribution by each of the various mechanisms of tolerance invoked through use of HSC transplantation and mixed chimerism , another major aspect that must be considered is the nature of the chimerism achieved, specifically whether it is transient or stable in nature. The majority of protocols utilized in small animal models (from extensive T cell depletion to costimulatory blockade) result in engraftment and establishment of long-term multilineage chimerism. In these situations, the continual contribution and differentiation of pluripotent HCSs into common lymphoid and myeloid progenitors ensure sustained input of donor-derived cells, facilitating both central and peripheral mechanisms of tolerance. However, in the translation of these small animal models into large animal models and the clinic, it has become evident that establishing stable chimerism with contribution from both host-derived and donor-derived HSCs is much more challenging: in most cases, recipients either fail to maintain donor chimerism for the long term (as determined by peripheral blood analysis by either flow cytometry or polymerase chain reaction (PCR), and lack of evidence for donor HSC in bone marrow samples), or they spontaneously convert to full-donor chimerism, with the attendant risk of GvHD.
With regards to the requirement of sustained donor hematopoiesis for establishment and maintenance of tolerance for organ transplantation, the current data in large animal and clinical trials do not yet provide clear insight into the necessity of the various mechanisms for optimal outcomes. While the initial studies described for combined HSC and kidney transplant described successful engraftment of donor HSCs and long-term hematopoiesis [43], these patients were being treated for both kidney failure and hematological disorders. However, further studies demonstrated that only transient chimerism was necessary for long-term kidney acceptance [27, 129], suggesting that peripheral mechanisms were sufficient and that the kidney may play an active role in promoting its acceptance. From a therapeutic perspective, this has the benefit of avoiding the risk of GvHD in patients, although recent studies have demonstrated the establishment of long-term donor chimerism in the absence of GvHD and concurrent acceptance of kidneys without immunosuppression [28].
Infusion of donor bone marrow cells has been included as part of the induction regimen for some cases from the outset of the clinical VCA era, with mixed evidence for effect. The recipient of the first face transplant received two donor bone marrow infusions, but chimerism was not detected; there was no evidence for modulation of anti-donor responses and comparable to protocols not including bone marrow , the recipient has experienced a number of rejection episodes despite conventional immunosuppression [2]. In contrast, a protocol including alemtuzumab induction and donor bone marrow infusion has been shown to have a positive effect, permitting acceptance of upper extremity transplants on significantly reduced maintenance immunosuppression (tacrolimus monotherapy) [14]. From a mechanistic perspective, as these patients have not received any conditioning that would likely facilitate engraftment of donor HSCs and the establishment of long-term chimerism, the infusion of donor marrow most likely is providing a window of donor-specific nonresponsiveness, mediated through either peripheral deletion of donor-reactive lymphocytes or induction of regulatory cells. However, in contrast to the kidney, as yet there is little evidence to suggest that VCAs will be able to contribute to their own long-term acceptance, and the short-term effects of bone marrow infusion may not be sufficient to ensure immunosuppression-free survival due to the inherent qualities of the VCA Fig. 15.4.


Fig. 15.4
Protocols achieving stable mixed chimerism result in the persisting contribution of donor cells to the thymus, and sustained clonal deletion. Protocols achieving transient or limited chimerism may require greater contribution from nondeletional mechanisms to maintain tolerance
Issues Specific to VCA Tolerance
VCA transplantation has only relatively recently entered substantial clinical application, and consequently, many well-accepted paradigms in solid organ transplantation remain to be specifically tested in VCA models. While it is likely that many of the basic principles of transplant acceptance and rejection will be shared, and protocols comparable to those sufficient for prevention of solid organ transplant rejection in clinical practice have achieved an impressively low rate of VCA loss to rejection [3], some aspects specific to VCA warrant further consideration and investigation.
The relative difficulty in achieving acceptance of skin across allogeneic barriers in comparison to kidney and other organ transplants is well established. Classically, this has been attributed to skin’s unique mode of transplantation (as a secondarily vascularized split or full-thickness skin graft), or to the presence of skin-specific alloantigens [130]. Presumably, the prolonged period of relative ischemia and subsequent ischemia–reperfusion injury following secondary establishment of circulation contribute to a local inflammatory milieu and presentation of donor antigen to the immune system in a strongly immunogenic fashion. However, it has been observed in both experimental models and clinical practice that the skin component of a primarily VCA is particularly susceptible to acute rejection episodes, suggesting a unique intrinsic factor of the skin.
It is now well established that skin provides more than a simple mechanical barrier in the protection against pathogens, and is a site of significant immunologic activity. The identification of Langerhan’s cells as professional APCs within the epidermis [131], and the ability of epidermal cells to stimulate T cells directly [132] suggested a model where skin can serve as a site of immune sampling and effector immune function. Elucidation of a network of multiple immunologically active cell types within skin suggests that in fact the “skin immune system” may be considered another unique and distinct component of the overall immune system [133].
The scale of the skin immune system has only recently begun to be appreciated, as recognition that the skin of a normal, healthy adult contains approximately 1 × 106 T cells/cm2;almost twice the number circulating in blood. These T cells express the skin-homing addressins cutaneous lymphocyte-associated antigen (CLA) and chemokine (C-C motif) receptor (CCR)4, and the majority lack expression of CD62L and CCR4, a phenotype consistent with effector memory T cells. The presence of such a significant population of T effector memory (TEM) cells in normal skin stands in contrast to the conventional model where TEM remain primarily in the circulation until recruited to the tissues by inflammation . Furthermore, these skin-resident TEM cells persist long term without recirculation, and appear to provide global cutaneous immunity which accumulates over time [134, 135].
Tregs have also been identified in human skin, where they represent between 5 and 10 % of the resident T cell population [136, 137]. Interestingly, expansion of cutaneous Tregs has recently been demonstrated in response to corticosteroid treatment, through upregulation of TGF-β secretion by Langerhans’ cells [138], suggesting a mechanism through which topical application of clobetasol may act in the treatment of acute rejection episodes in VCA patients.
While skin represents a well-established challenge to transplant tolerance induction, both when transplanted in isolation and more recently as a component of VCA, there is evidence to suggest that VCAs may in fact be less immunogenic than the sum of their parts [139]. One frequent outcome in large animal studies has been the induction of split tolerance, with long-term acceptance of musculoskeletal components of limb allografts but rejection of skin, across both minor histocompatibility antigen (miHA) and MHC barriers. In some cases, rejection has been targeted specifically at epidermis with maintenance of viable dermal tissue [140, 141].
The establishment of mixed chimerism by HSC transplantation, and the association of chimerism and tolerance have been extensively discussed. Clearly, the conditioning regimens necessary to achieve engraftment of donor HSCs retain some degree of toxicity and morbidity, despite considerable advances in the development of reduced intensity regimens. It has been suggested that the vascularized BMT (VBMT) may offer benefits over cellular BMT in this regard, by providing a source of donor HSCs pre-engrafted within appropriate cellular architecture.
Much of the data concerning the immunologic role of VBMT has been collected from experimental models of hind-limb transplantation in rats [142, 143]. The utility of VBMT as a source of functional hematopoiesis was demonstrated by the complete repopulation with donor cells of bone marrow and secondary lymphoid organs in lethally irradiated recipients [144, 145]. Subsequent studies demonstrated induction of mixed chimerism , long-term-immunosuppression-free survival of the allograft, and in vitro evidence of tolerance of donor antigen in a parental to F1 hybrid setting [146]. More recently, a number of small animal models of VBMT-containing VCA have been introduced, permitting study of both immunologic aspects of these procedures and functional responses to transplantation and various conditioning and immunosuppressive regimens [147].
VCAs containing a vascularized bone marrow compartment have also been studied in large animal systems, including heterotopic hind-limb models in both porcine [140, 141] and canine systems [122], and partial face allografts in nonhuman primates [148]. In a series of studies in MGH miniature swine, tolerance of musculoskeletal components, but not skin, was observed in untreated recipients of MHC-matched, minor antigen-mismatched limb transplants. In contrast to some small animal studies, evidence for sustained donor hematopoiesis was not observed either in peripheral blood or in the recipient bone marrow compartment and by 48 weeks post transplant donor cells could no longer be isolated from the donor marrow compartment, which had been repopulated with recipient origin cells [149
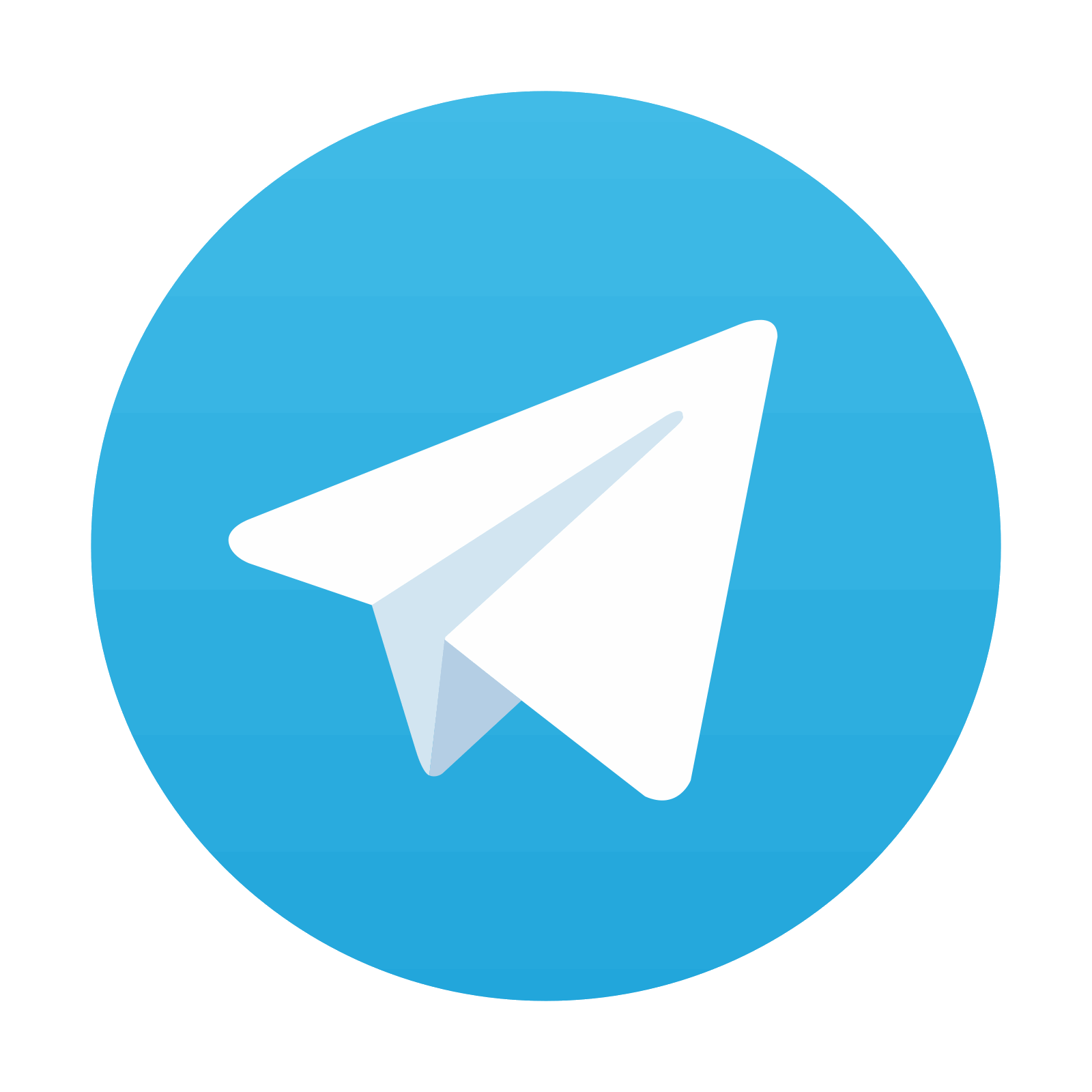
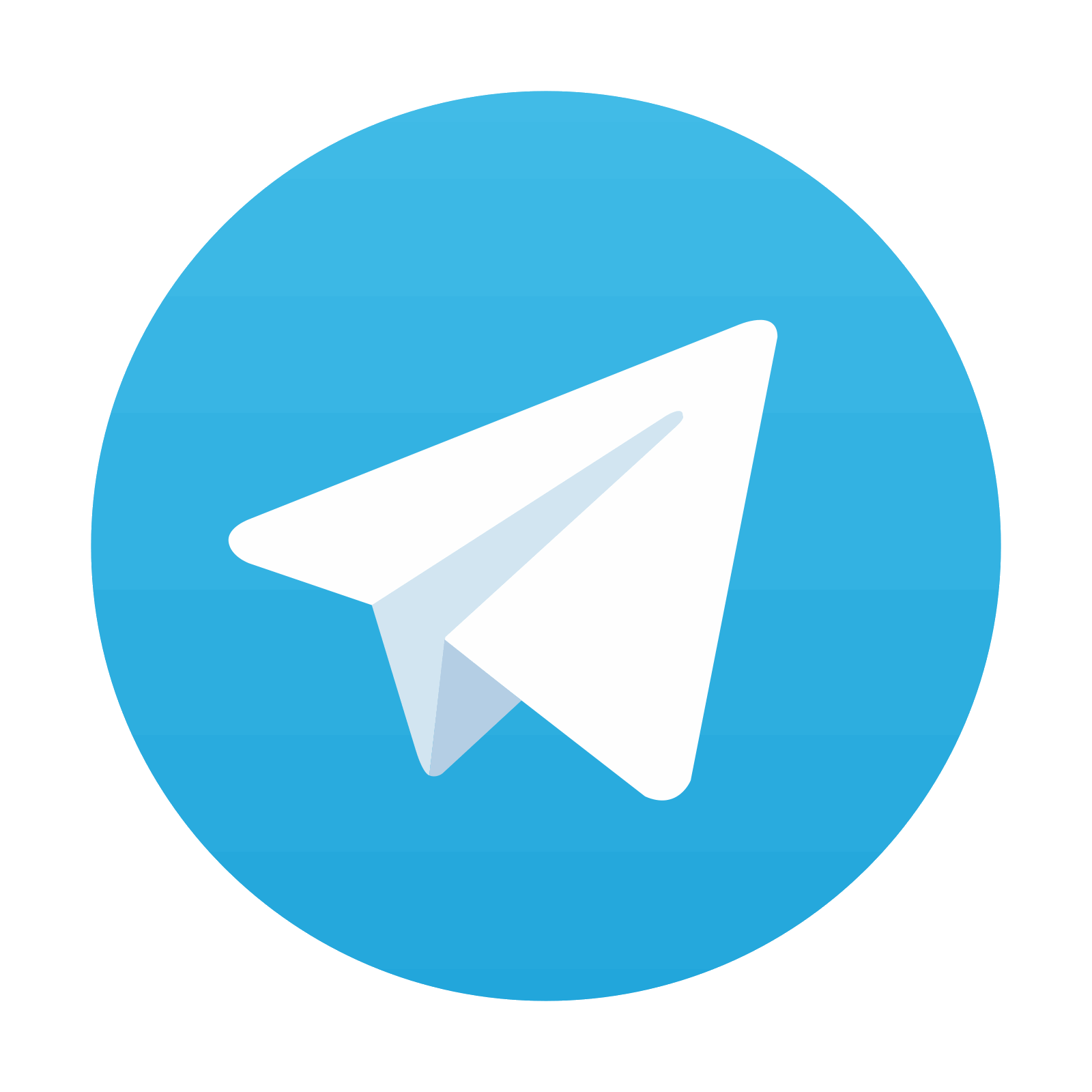
Stay updated, free articles. Join our Telegram channel
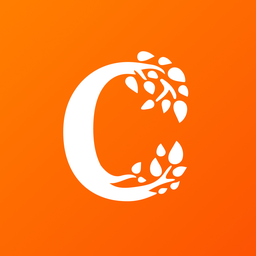
Full access? Get Clinical Tree
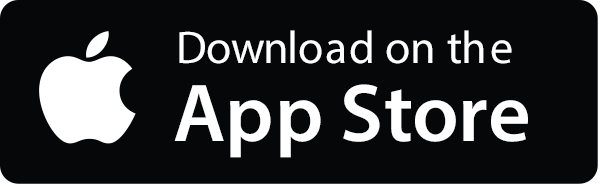
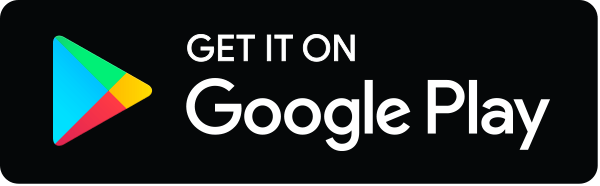