Fig. 20.1
Preparation of fibrin conduits. a The fibrin conduit (Tisseel) with stainless steel wire inserted in the lumen as a spacer while the conduit is store before implantation. b The fibrin conduit shows an intact lumen after removal of the stainless steel wire. c The fibrin conduits are stored in DMEM medium before being seeded with regenerative cells. d A fibrin conduit implanted in vivo to repair a rat sciatic nerve injury. DMEM Dulbecco modified Eagle’s medium
However, the use of autologous cultured SC for the treatment of acute injuries may be impractical, owing to the technical difficulties, and time required, in harvesting and expanding such cells. In this scenario, the ideal ‘transplantable cells’ should be easily accessible, capable of rapid expansion in culture, immunologically inert, capable of long-term survival and integration in the host tissue and amenable to stable transfection and expression of exogenous genes [24]. In a search for such cells, attention has been drawn to the possible use of stem cells. Stem cells are distinguished from other cell types by two important characteristics. First, they are unspecialized cells capable of renewing themselves through cell division, sometimes after long periods of inactivity. Second, under certain physiologic or experimental conditions, they can be induced to become tissue- or organ-specific cells with special functions.
In this context, MSC seem to be a promising option for cellular therapy, as they are relatively easy to harvest, higher aliquots available and grow faster if culture expanded. MSC have originally been harvested from bone marrow mesenchymal stem cells (BM-MSC), and so for many years, yet other MSC sources have been found such as placenta, skin, thymus, umbilical cord, amniotic fluid and Wharton’s jelly, including the abundant and easy accessible subcutaneous fat tissue, the so-called adipose tissue-derived stem cells (ADSC) [25]. ADSC are of great interest because of recent studies showing they might have similar phenotypic, genetic and differentiation characteristics to BM-MSC but are obviously easier to harvest and more abundantly available [26]. Therefore, herein we focus on the application of both BM-MSC and ADSC to promote nerve regeneration after PNI.
Cellular Therapy for PNR
Basic Biology of Stem Cells
The definition of a stem cell describes it as a clonogenic cell capable of self-renewal and multilineage differentiation [27]. A number of criteria have been proposed to help identify a stem cell. These dictate that the cell must be (i) undifferentiated (i.e. lacking a tissue-specific differentiation marker), (ii) capable of proliferation, (iii) self-renewable, (iv) able to produce a large number of differentiated functional progeny and (v) able to regenerate tissue after injury [28]. The proportion and activity of stem cells within adult tissue depends on tissue type, i.e. depending on whether the tissue is renewing, e.g. intestinal epithelium, or static, e.g. the central nervous system [29]. With such diversity in stem cell activity within different tissues, it is obviously important to understand the regulatory mechanisms which control the two key properties of stem cells, i.e. self-renewal and differentiation which is the most fundamental property of stem cells. Briefly, under steady-state conditions, the stem cell pool remains constant, but following injury or during disease, it can expand rapidly. The haematopoietic system displays regenerative capacity more dramatically than any other tissue, as huge numbers of cells are continuously produced throughout life, a property which would be impossible if there was a fixed, and hence depletable, number of progenitors of any particular haematopoietic subset. Cells mobilized from bone marrow, peripheral blood and cord blood are capable of re-establishing the entire haematopoietic compartment following complete ablation, as is seen following cytotoxic therapy for haematological malignancies; indeed, it is this property which has been exploited to successfully treat such conditions [30]. Embryonic stem cells can be propagated in culture [31]; however, obtaining such cells presents practical, ethical and legislative difficulties [32, 33]. The identification, isolation and expansion of truly multipotent adult-derived stem cells remain problematic. Toma et al. [34] fairly recently described the isolation and expansion of multipotent stem cells from skin; however, it was not possible to determine the origin of the cells with absolute certainty. The problem of isolating clonal stem cells from adult tissues is probably the greatest obstacle faced in this field [35, 36].
There are a number of paradigms to describe regulatory mechanisms in stem cell division and self-renewal. The mechanistic of stem cell expansion and division have been described as either ‘asymmetric’ or ‘symmetric’. In asymmetric division, each stem cell divides into a daughter stem cell and a differentiated cell, and thus, there is no change in the total number of stem cells in the stem cell pool. A number of genes have been implicated in the control of asymmetric cell kinetics, such as the p53 tumour-suppressor gene [37] and the p21 cyclin-dependent kinase inhibitor gene [38]. In symmetric division, half the time stem cells divide into either two daughter stem cells or two daughter differentiated progeny, leaving the steady-state number of stem cells constant, similar to the situation in asymmetric division [39]. A third model involves the role of the microenvironment. Depending on environmental cues, such as injury, a stem cell may produce two daughter cells, which will either remain as stem cells or differentiate, depending on the environment.
The control of self-renewal and differentiation is most probably influenced by extrinsic factors, i.e. the environment or niche, and intrinsic cellular factors. The environment influences the biochemical and morphological properties of stem cells. Stem cells adjust their properties according to their surroundings and select specific lineages according to the cues they receive from their niche [40]. Extrinsic factors can be divided into cell–cell interactions, locally secreted factors and the extracellular matrix. Cell–cell interactions mediated by integral membrane proteins have been shown to influence the maintenance of self-renewing potential and differentiation. For example, Notch-related receptors activated by ligands only found on neighbouring cells maintain stem cells in an undifferentiated and self-renewing state, and upon removal of the ligand, cells begin to differentiate [41, 42]. Locally secreted factors or growth factors play an important role in regulating stem cell proliferation within the niche. Growth factors, such as epidermal growth factor and basic fibroblast growth factor, stimulate stem cell proliferation [43, 44]. They may act selectively on specific progenitors after stem cell division or instructively by stimulating a specific group of stem cells to differentiate into a specific progeny [45, 46]. Within the extracellular matrix, cell adhesion is mediated by cellular integrin expression. The extracellular matrix can modulate the local concentration of growth factors and hence indirectly influence stem cell proliferation and differentiation within the stem cell niche [47]. Extracellular matrix proteins influence the expression of integrins, alterations of which can lead to departure of a cell from the stem cell niche towards differentiation [48].
Intrinsic factors determining self-renewal and division exist. Under similar conditions, it has been observed that sub-populations of haematopoietic stem cells have different capacities for self-renewal depending on telomerase activity, with increased telomerase expression correlating with an increased capacity for self-renewal [49]. Maintenance of the undifferentiated state may be determined by the asymmetric inheritance of certain proteins which influence gene expression; for example, the nuclear protein pharynx and intestine in excess-1 (PIE-1) inhibits embryonic genes which are responsible for somatic lineage differentiation [50]. The role in vivo of asymmetric kinetic genes may be of fundamental importance as intrinsic factors controlling self-renewal; however, it is believed the predominant form of self-renewal in mammals detectable so far is symmetric division [51]. Manipulation of asymmetric genes in vitro may enable great advancement in stem cell expansion [52]. The role of systemic cytokines and growth factors in stem cell regulation is less clear. Although a large number of growth factors have been identified that are mitogenic for haematopoietic stem cells such as granulocyte colony-stimulating factor [53], interleukin-1 (IL-1), IL-3 and IL-6 [54], leukaemia inhibitory factor [55] and basic fibroblast growth factor [56], long-range regulatory mechanisms for tissue-specific stem cells are unclear, as these cells are thought to be primarily responsive to local changes in the stem cell niche as their regenerative capacity is usually called upon in circumstances of local tissue damage. However, the control of differentiation is a complex event requiring exit of the cell from the undifferentiated state and entry into a determined developmental pathway. At present, cell determination is seen as a stochastic event initiated by intrinsic factors with an outcome biased by extrinsic factors. The challenge at present is to identify further intrinsic and extrinsic regulatory mechanisms which determine stem cell fate and to define the relationship between these systems. Developmentally, the components of the peripheral nervous system originate from the neural crest. In looking for alternatives to SC transplantation in bioengineered conduits, it is useful to focus on utilizing neural progenitor cells in PNR.
Sources of Stem Cells for PNR
Emphasis has been placed on exploring stem or progenitor cells that are easily accessible, rapidly expandable in culture, capable of survival and integration within the host tissue and amenable to stable transfection and expression of exogenous genes. Table 20.1 summarizes some of the studies conducted on nerve repair mechanisms to date. Embryonic neural stem cells have been used to repair nerve injuries with demonstration of regenerative success [57, 58, 59] but suffer the drawback of being somewhat difficult to obtain. On the other hand, adult stem cells (ASC) have the advantage of being available from relatively non-invasive, autologous harvest methods, and are likely the most promising choice for the majority of clinical nerve injuries. Bone marrow stromal cells have attracted the attention of several groups interested in cellular strategies to supplement nerve repair [60–63]. The mesenchymal stem cells are harvested from the long bones, and when placed in culture medium containing the appropriate cytokine cocktail, transdifferentiate into an adherent SC-like phenotype expressing S100 protein, glial fibrillary acid protein (GFAP) and p75. Several studies have been used with artificial conduits and cellular grafts, where they have contributed to improved electrophysiological, morphometric, and/or behavioural recovery outcomes versus vehicle controls. Although their potential to produce functional myelin in vivo has been questioned,[64] others have shown that the BM-MSC-derived SC are at very least capable of myelinating cultured PC12 cells in vitro further highlighting their therapeutic potential.
Table 20.1
Selected studies of transplanted stem cells for peripheral nerve repair
Cell source/type | Authors and year | Donor/host animal | No. of cells injected | Delivery method | Cell survival time | % Survival | Phenotype | Regenerative advantage conferred over vehicle |
---|---|---|---|---|---|---|---|---|
Bone marrow aspirate/mesenchymal stem cell | Hu et al. 2007 | Rhesus monkey in | 2 × 107 | Proximal/distal side of acellular allograft | ND | ND | ND | ↑ No. of NF + axons, improved CMAP amp/latency |
– | Keilhoff et al. 200625 | Wistar rat | 2 × 106/ml | Devitalized muscle conduits | 6 wks | ND | MBP+, bipolar morphology in predifferentiated cells only | ↑ No. of myelinated fibers; faster return of thermosensitivity |
– | Chen et al. 2007 | Sprague-Dawley rat | 106 cells | In gelatin w/in lumen of silicone tube; 15-mm gap | Unable to detect due to label loss | ND | Express neurotrophins; not P0, PMP22 | Improved SFI, improved CMAP amp/latency |
– | Dezawa et al. 2001 | Wistar rat | 1–2 × 107 cells/ml | In Matrigel, w/in hollow fibers; 15-mm gap | 3 wks | MD | MAG+; produced myelin | ↑ Axonal outgrowth achieved w/ predifferentiated cells |
– | Zhang et al. 2004 | Sprague-Dawley rat | 107 | Microinjected into crush-injured sciatic nerve | Up to 3 wks | ND | Limited expression of GFAP, S100, p75 | ND |
– | Shimizu et al. 2007 | Human/Wistar rat | 1–2 × 106 cells/ml | In Matrigel, w/in transpermeable tube; 10-mm gap | 3 wks | < 12.6 ± 2.98 % of all MAG+ SCs | MAG+; enveloped re-generating axons; many phagocytozed | Slight ↑ SFI conferred by transdifferentiated cells over |
– | Tohill and Terenghi 2004 | Sprague-Dawley rat | 8 × 106 cells/ml | w/in PHB conduits; 10-mm gap | Up to 15 days | ND | Some differentiated to S100+ SCs | Naive ↑ outgrowth |
C17.2 neonatal cerebellar granule cells ± overexpression of GDNF | Heine et al. 2004 | Mouse cell line/Sprague-Dawley rat | 5 × 105 cells | Subepineural injection into chronically denervated nerve | Up to 4 mos | 0.5–1 % | Most remained distal to repair site, very few GFAP or NF+; mesenchymal tumour | ↑ No. of axons, improved CMAP amp/latency |
Hippocampal E17 neuronal progentitor cells | Murakami et al. 2003 | Fischer rat | 105 cells | In collagen gel w/ in silicone tube; 15-mm gap | Up to 10 wks | ND | some cells positive for S100/p75 | Superior electrophysiological recovery |
E11 DRG/boundary cap neural crest stem cells | Aquino et al. 2006 | Rosa 26 mouse (lac-Z+)/Sprague-Dawley rat | 4 × 103 cells | Intact nerve; cultured in 12-mm silicone tube & implanted in nerve gap | Up to 90 days; only predifferentiated cells survived | ND | 69.7–94.6 % GFAP+ after 13 & 60 days, respectively; MBP+ transplanted cells ensheathed axons in tube | ND |
Neonatal skin/neural crestlike precursors | Marchesi et al. 2007 | Wistar rat | 106 cells | In PBS in lumen of collagen guide; 16-mm gap | Up to 2 mos | 25–38 % | 4.5 % S100+, 6.1 % GFAP+ | Improved CMAP, SFI, no. of myelinated fibres |
– | McKenzie et al., 2006 | Rodent or human/shiverer mouse | 1–4 × 105 cells | Microinjected distal to crush injury | ³ 6 wks | ~6.5 % | 70.4 % of transplanted cells MBP+, associated w/ NFM+ axons | MBP positive myelin on shiverer axons |
Vibrissal follicles | Amoh et al. 2005 | C57/B6-GFP/C57/B6 mouse | ND | Transplanted btwn severed sciatic/tibial nerve stumps | Detected after 2 mos | ND | GFAP+; envelop β III tubulin+ axons | Improved SFI, contraction of gastrocnemius |
Amniotic fluid/mesenchymal stem cells | Murakami et al. 2003 | Sprague-Dawley rat | 1.5 × 104 cells | In fibrin glue around crushed sciatic nerve | Up to 10 days, none at 4 wks | ND | NT-3 and CNTF+; no expression of GFAP/S100β | Motor function recovery, improved CMAP |
Neural Progenitor Cells
The transplantation of primary SC has been shown to improve nerve regeneration through bioengineered conduits . However, this approach has a number of pragmatic difficulties if it is to be applied to the clinical setting, the greatest of which is accessing a source of primary SC. Such cells would have to be autologous to prevent graft-versus-host reactions, as the use of immunosuppressants is not considered ethically acceptable in the case of nerve injury, on account of their systemic side effects. Hence, transplantable cells would have to be donor derived, being harvested and expanded from the individual with nerve injury prior to transplantation of the conduit. Primary human SC are technically difficult to purify, culture and expand to the numbers’ required optimal regeneration inferred from experimental studies in a rat model. The time frame of culture to appropriate numbers can be up to 10 weeks. Such a delay in performing a primary nerve repair following injury would be deleterious to clinical outcome. Indeed, it has been shown that the longer the time lag between injury and repair, the greater the neuronal cell death in the dorsal root ganglia and therefore a reduced potential for recovery [65]. To circumvent these practical problems of SC transplantation, attention has been turned towards stem and progenitor cell transplantation. Possible candidates for peripheral nerve transplantation are neural progenitor cells, olfactory ensheathing cells (OECs) and MSC-derived cells. There are a limited number of reports describing the use of stem and progenitor cells in peripheral nerve transplantation. SC are derived from the neural crest of the neuroectoderm and differentiate and migrate into the peripheral nervous system with development [66]. The use of neurally derived progenitors from the fetal rat hippocampus to seed a conduit spanning a 15-mm gap in the rat sciatic nerve has been described. Significant morphological and functional evidence of regeneration, with integration and differentiation of transplanted neural progenitors into SC-like cells, was reported [67]. Neural stem cells have been identified in the adult central nervous system [68]. Such adult-derived stem cells and those isolated from fetal tissues have been successfully propagated and differentiated in vitro into all major cell types of the nervous system [69]. The use of fetal or adult-derived neural progenitors fails to avoid the problems encountered with SC, i.e. the source of donor tissue and the time taken to expand such cells.
Bone Marrow Stromal Cells
Until recently, dogma dictated that organ-specific stem cells were restricted to differentiate only into cell types from the tissue from which they originate. Unlike embryonic cells, organ-specific stem cells were believed to have lost their capacity to generate other somatic lineages. However, recent reports have suggested that stem cells from one tissue can cross lineage boundaries to differentiate into cells of other lineages either in vitro or in vivo after transplantation. This plasticity, or ability for cells to transdifferentiate, has aroused great interest for its therapeutic potential in tissue engineering. A promising candidate to display such plasticity is the bone marrow stromal cell.
Bone marrow contains two distinct populations of progenitor cells: haematopoietic progenitors and bone marrow stromal progenitors. Bone marrow stroma has been identified as the site of origin for mesenchymal progenitors for bone, cartilage, tendon, adipose tissue and muscle [70]. In addition, the stroma has been identified as a supportive tissue for the haematopoietic system, enhancing cytokine-induced proliferation of haematopoietic precursors [71]. A semantic issue confuses the description of the progenitor cells of the stroma, as they have been defined historically as colony-forming unit fibroblasts, marrow stromal fibroblasts, marrow stromal cells (MSCs), mesenchymal stem cells and mesenchymal progenitor cells. Unless such cells can be shown to be clonogenic and undifferentiated prior to their use in experimental studies, the putative use of the term ‘stem cell’ should be avoided. MSCs are easily accessible through the aspiration of the bone marrow cavity. They readily adhere in tissue culture in comparison with their non-adherent haematopoietic counterparts [72]. A number of mitogenic factors have been identified which stimulate colony formation and proliferation such as platelet-derived growth factor, epidermal growth factor, basic fibroblast growth factor, transforming growth factor b and insulin-like growth factor-I (IGF1) [73]. Bone marrow stromal cells have been shown to be inherently heterogeneous in terms of growth kinetics, morphology and phenotype. This may in part be due to the fact that the actual number of true stem cells is small, being estimated at 2–5 per 106 mononuclear cells, with the majority of cell growth arising from expanding differentiated colonies under mitogenic influence. With the development of specific antibodies such as STRO-1 [74] and characterization (CD45-ve, CD34-ve, CD105 + ve, CD73 + ve) [75] for human mesenchymal stem cells, their expansion and properties can be studied and defined with more certainty.
Interestingly, MSCs differentiate according to a hierarchical paradigm into osteoblastic, chondroblastic and adipocytic progenitors [76]. Orthodox plasticity between these lineages has been reported from adipocytic and chondrocytic lineages to osteogenic differentiation [77, 78]. Recent reports have described unorthodox plasticity of haematopoietic progenitors [79, 80] and bone marrow stromal cells in that they have been shown to cross oligolineage boundaries which were previously thought to be uncrossable. The potential of MSCs to transdifferentiate from mesenchymal lineages has aroused great interest. An early report by Ferrari et al. [81] described the migration of labelled MSCs to areas of damaged skeletal muscle. Transdifferentiation was detected, owing to the stromal cell expression of myogenic markers and their participation in the regeneration of damaged muscle fibres. Gojo et al. [82] demonstrated mesenchymal differentiation into cardiomyocytes, endothelial cells and smooth muscle cells following direct injection into adult heart; it has also been shown that these cells will migrate to zones of myocardial injury following systemic delivery [83], although neither group of authors described any functional improvement. Jiang et al. [84] identified marrow-derived cells in all somatic lineages in chimeric mice following injection of labelled cells into 3–5-day-old blastocysts and engraftment into many adult tissues following systemic infusion. There is evidence that MSCs are capable of neuronal antigen expression in vitro [85–87] and in vivo [88, 89]. They have been shown to differentiate into astrocytes following direct transplantation into the rodent brain [88]. Studies have also reported the ability of such cells to transdifferentiate into cells displaying a characterization similar to that of neuroectodermal cells [88, 89]. Akiyama et al. [90] described re-myelination of spinal cord lesions following intravenous delivery of MSCs, and Hofstetter et al. [91] showed that local delivery of MSCs at the site of spinal cord injury was associated with the formation of neurofilament bundles at the interface between scar tissue and graft.
Similarities and Differences of BM-MSC and ADSC
MSC from different sources seem to have overlapping characteristics but also differences in their phenotype (e.g. cytokine secretion properties and receptor profiles) as well as differentiation and expansion potential [92–95]. BM-MSC have been studied for many decades now and are the best-known and characterized type of MSC [96–98]. In animal studies as well as clinical trials, BM-MSC have shown beneficial outcomes in a variety of diseases ranging from ischemic and inflammatory disorders [99–101], through wound healing [102] and lung diseases, to tissue engineering and regenerative medicine, including nerve regeneration [103]. BM-MSC are easily aspirated from bone marrow, but associated with discomfort (pain) and potential morbidities after bone marrow punction.
ADSC bear some appealing characteristics in respect to BM-MSC and are thus rapidly gaining the interest of researchers. The main advantages are the ease of harvesting, higher availability of subcutaneous tissue, higher cell aliquots [104, 105], less harvest-site morbidity and the apparently higher expansion potential [106, 107], suggesting them as an ideal candidate for clinical application in the typical scenario of PNI.
There is plenty of subcutaneous fat tissue in humans which can deliver already high cell numbers after harvesting, shortening the culture time span required to achieve the desired cell aliquots [108]. In fact, since during culture MSC lose cytokines and receptors with increasing passage number, influencing their differentiation and homing capabilities (due to increasing cell size and change in receptor and chemokine profile), shorter culture times seem to be beneficial for clinical application [109, 110]. However, the beneficial effect of ADSC, compared to BM-MSC, has to be further investigated.
BM-MSC have been shown to decrease their expansion capabilities after passage 3–4 [111], showing signs of senescence, whereas ADSC do not, which together with the fact that bone marrow contains fewer MSC highlights the superiority of ADSC for achieving high cell yields shortly.
Some authors suggested that MSC from different sources may have different potential of differentiation towards the mesenchymal lineages [112], which could also be true for non-mesenchymal lineages as for neural tissues, whereas other groups found no significant differences between their differentiation potential [113]. Furthermore, it has been shown that BM-MSC and ADSC might differ in their homing capability [114].
Mesenchymal Stem Cells in the Peripheral Nervous System
Two recent reports have described the use of MSC transplantation in models of PNI. Dezawa et al. [115] described the in vitro expression of the glial cell markers p75 and S100 by rat MSC following exposure to a cocktail of growth factors, and integration of such cells in the regenerating growth cone upon transplantation into a blind-ending tube grafted to the proximal stump of the rat sciatic nerve. Cuevas et al. [116] described the migration and differentiation of MSCs following the injection of cultured undifferentiated cells into the site of a sciatic nerve axotomy repair. In our laboratory, we have studied rat marrow stromal cell differentiation in vitro and in vivo following transplantation into a model of PNI. Following in vitro exposure of MSCs to glial growth factor, a powerful SC mitogen [117] which has also been shown to stimulate neural crest stem cells to differentiate into SC, MSCs were found to exhibit glial cell immunoreactivity to markers, including GFAP and S100. In addition, a small percentage of cells were also found to exhibit some morphological characteristics of SC. We have studied the effect of transplanting such glial-differentiated MSCs into a rat model of PNI and have found that they can confer a beneficial effect on SC growth within regenerating nerves, indicating either a stimulatory or a supportive role.
Immunotolerance to Allogeneic Stem Cells
An important problem which faces all studies of transplantation is that of host rejection of transplanted tissue. In the clinical setting of organ transplantation, this problem is overcome with the use of immunosuppressants, with the benefits of a functioning heart, lung, liver or kidney outweighing the potentially serious systemic side effects of immunosuppression, such as susceptibility to opportunistic infection and skin cancer. A number of experimental reports have shown that there is an unexpected and fortuitous level of immune tolerance to transplanted stem and progenitor cells. MSCs have been shown to block the proliferation of allogeneic T cells in vitro and prolong skin graft survival in vivo [118]. Saito et al. [119] demonstrated immune tolerance to xenogeneic cells following transplantation in addition to retaining their ability to engraft and differentiate. The ability of MSCs to induce tolerance may be due to cytokine (such as transforming growth factor b) secretion or due to their phenotypical immaturity, as they lack major histocompatibility complex (MHC) class II and other T cell stimulatory surface proteins [120, 121]. With respect to studies in the nervous system, Hori et al. [122] have shown that central nervous system progenitor cells may not express MHC class I and II in vitro and that no short-term evidence of rejection is seen after allogeneic transplantation within the central nervous system. These observations are seemingly a local effect of stem cell transplantation and are distinct from the recognized phenomenon that infusion of bone marrow donor cells can improve allograft survival and reduce the need for short-term immunosuppression. Such macrochimeric models require significant host conditioning, such as central and peripheral T cell depletion [123, 124], prior to transplantation.
One problem which may be faced when considering host tolerance to progenitor cell allografts is the consequence of the up-regulation of immunoreactive surface markers following in vitro or in vivo differentiation due to the effects of growth factors or cytokines. Such effects may offset the benefits of immune tolerance to immature cells. MSCs might be used as primary engrafting cells for differentiation into cells of a de novo tissue, and they might also be used as cellular platforms for transgene expression for the production growth factors or cytokines which could ameliorate the immune response to more differentiated and functionally mature allogeneic cells [125, 126].
In Vitro Studies
Isolation of Stem Cells
Bone marrow-derived mesenchymal stem cells can be harvested from femoral bones [34] and cultured in mesenchymal cell growth medium (modified Eagle’s medium, MEM) supplemented with 10 % fetal bovine serum (FBS; v/v and 1 % penicillin streptomycin). Briefly, a 21-guage needle can be used to run the medium in and out of bone to get the cell suspension. The resulting cell suspension is triturated, filtered through a 70-mm Falcon filter and centrifuged for 10 min at 600 g. The supernatant is aspirated, the cell bolus re-suspended in mesenchymal cell growth medium and the cells plated in 75-cm2 tissue culture flasks and incubated in 5 % CO2
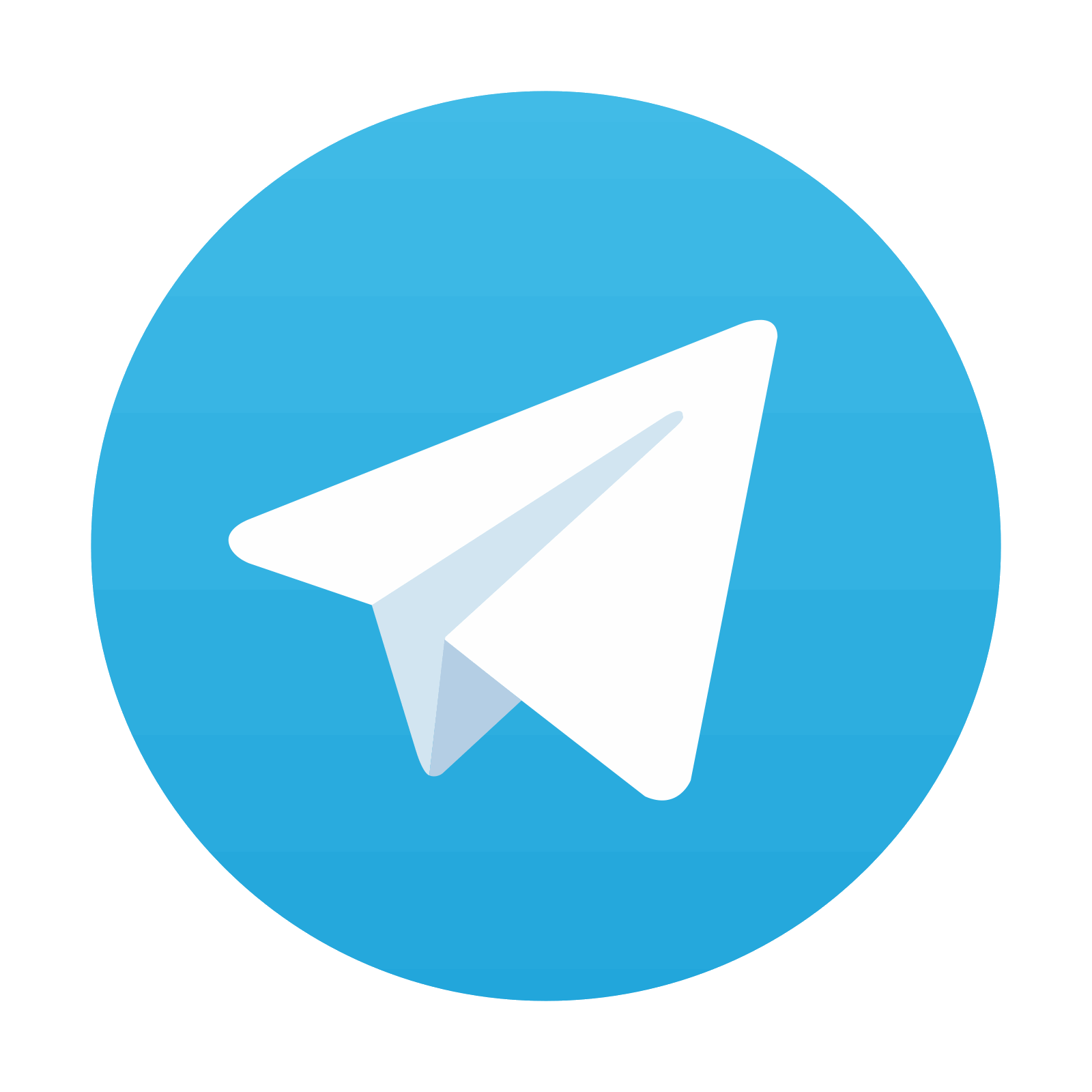
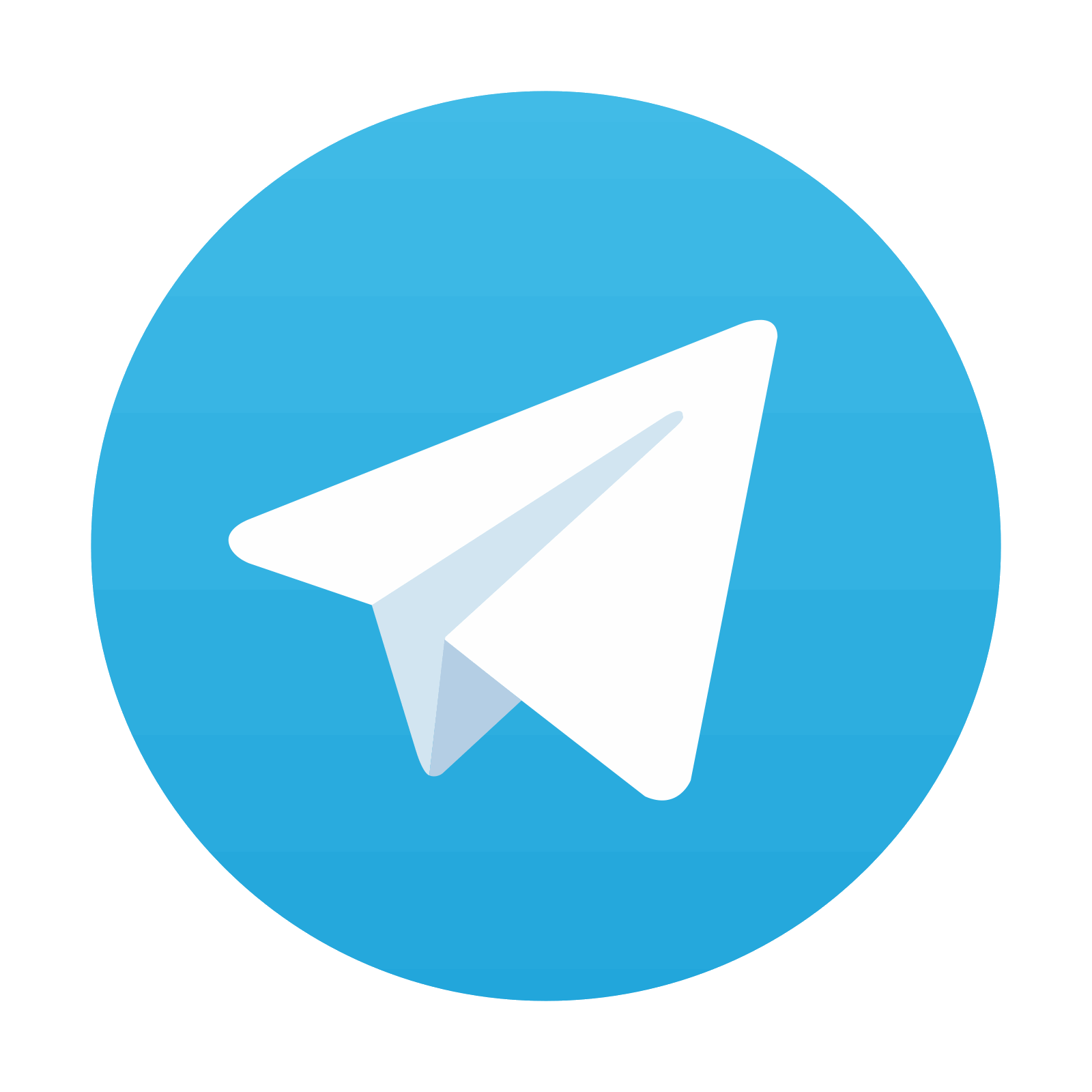
Stay updated, free articles. Join our Telegram channel
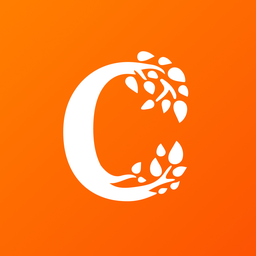
Full access? Get Clinical Tree
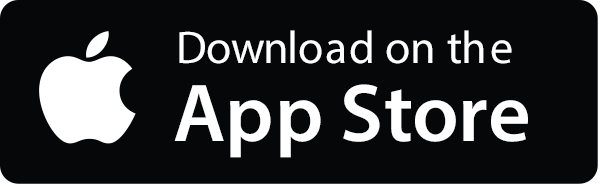
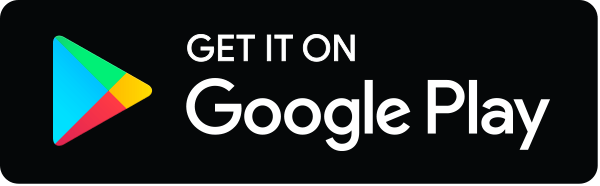