Fig. 17.1
Regenerative and immunomodulatory properties of mesenchymal stem cells (MSCs). MSCs help define and maintain the stem cell niche in the bone marrow where they undergo self-renewal. They are thought to function in this compartment by maintaining hematopoietic stem cells (HSCs) in a quiescent state, regulating the trafficking of both primitive and more differentiated leukocytes, and modulating sinusoidal endothelial cell function. Although largely unknown, they likely have similar functions in nonbone marrow stem cell niches in peripheral tissues. In addition to this supportive and regulatory function, MSCs are identified by their regenerative capacity to differentiate into different tissues of mesenchymal origin. Furthermore, there is evidence to suggest that transdifferentiation into nonmesodermal derivatives is also a possibility. In contrast to their regenerative properties, MSC have recently been identified as powerful immunomodulatory cells, with numerous functions which suppress both the innate and adaptive immune responses . This function is critical for their potential use in VCA. (VCA vascularized composite allotransplantation)
Mechanisms of MSC-Mediated Modulation of the Immune System
The biological response to allografts is a process that involves both the innate and adaptive components of the immune system. Modern biology has demonstrated multiple overlapping mechanisms in which both the innate and adaptive immune systems potentiate rejection. As a process, the cascade of allograft rejection is initiated at the time of reperfusion, initially fueled by inflammation related to IRI, and ultimately executed by immunologic attack of the graft vasculature and underlying parenchyma. Strategies to encourage graft tolerance have focused on not only reducing IRI but also reducing the immunologic sensitivity of the recipient’s immune system. To this effect, there has been a growing body of literature supporting the MSC’s integral role in promoting transplant tolerance [12, 27, 48, 53, 81, 109–112, 116, 118]. In both ischemia- and immunologic-related injury, MSCs have significant therapeutic potential from their ability to secrete soluble factors and communicate with surrounding cells to modulate the local inflammatory microenvironment. These dynamic interactions have multipronged effects that can be exploited for use in VCA.
The Adaptive Immune System and MSCs
Although it has been almost 30 years since the hypothesis that marrow-derived stem cells could promote tolerance when transplanted into a mismatched recipient [44], only recently have MSCs been shown as a critical mediator of transplant tolerance. In the context of the adaptive immune system, MSCs have been shown to specifically inhibit T cell proliferation in vitro [56, 57, 93], and more importantly, have the potential to prolong allogeneic skin engraftment in nonhuman primates in vivo [3]. When we look at specific mediators of allograft rejection, cytotoxic T cells (CTLs) represent effector cells of the adaptive immune system and are powerful mediators of parenchymal damage and inflammation. In experimental models of allograft rejection, CTLs lose their lytic abilities in the presence of MSCs [90, 101]. While the exact molecular mechanisms are still a matter of debate and vary between experimental models, most studies agree that MSCs do not constitutively exert their immunomodulatory functions. Rather, they are induced into that role through frequent cross-talk interactions with surrounding T lymphocytes and inflammatory cytokines within the allograft environment. Several specific factors have been hypothesized to contribute to this phenomena, including transforming growth factor (TGF)-β, hepatocyte growth factor [19], prostaglandin E2 (PGE-2; [1]), as well as nitric oxide [97] and indoleamine 2,3 dioxygenase [56].
PGE-2 is a small, short-acting lipid-signaling molecule that has long been linked to inflammation. The pathways for prostaglandin synthesis are mediated by the cyclooxygenase enzyme (COX-1 and COX-2), which produces PGE-2 from arachidonic acid. MSCs have been shown to have baseline expression of COX-2 [25] that is significantly increased in the presence of inflammatory cytokines such as interferon (IFNγ), TNFα, and interleukin 6 (IL-6). MSC-elaborated PGE-2 has been linked to suppressor T-cell activation in vitro and in vivo [1, 80]. Beyond T-cell activation, MSC-derived PGE-2 has been shown to inhibit production of T helper (Th)17 T-cell populations, a cell population that impairs peripheral tolerance to allografts [40].
Nitric oxide (NO) is a rapidly diffusing gaseous and bioactive molecule, particularly in the context of vascular biology and transplant immunology. NO production is catalyzed by the nitric oxide synthases (NOS) by a variety of cell types, including endothelial cells and MSCs. NO exerts its effects locally at high concentrations, often induced by inflammatory conditions. MSC-derived NO can suppress T-cell proliferation and promote T cell apoptosis [93]. In an elegant study in a murine system [93], MSCs have been shown to chemoattract T cells using various chemokines, and once in proximity, they release NO to exert local immunosuppression. In experimental models of graft-versus-host disease (GvHD), MSCs produce nitric oxide (NO) in a dose-dependent manner in response to interactions with CD4+ or CD8+ T cells, facilitating their immunomodulatory effects [93, 97].
Of recent interest, the tryptophan-metabolizing enzyme, indoleamine 2,3 dioxygenase (IDO) has been implicated in the suppression of T cell proliferation and apoptosis of activated T cells [48, 81]. Tryptophan is an essential amino acid required for T cell proliferation and is depleted through expression of the tryptophan-metabolizing enzyme, indoleamine 2,3 dioxygenase. Via local tryptophan depletion and subsequent production of pro-apoptotic downstream metabolites, IDO provides the common basis for tolerance induction in a variety of physiologic conditions including pregnancy, autoimmunity, tumor immunosurveillance, and transplantation [9]. MSC expression of IDO can be induced via stimulation from IFNγ, or through toll-like-receptor (TLR) 3 and TLR4 ligands. Moreover, MSC-derived IDO has been shown to “reeducate” immune cells towards a more immunosuppressive phenotype through promotion of a Th1–Th2 switch, altering the helper T cell balance and overall adaptive immune response. MSCs also modulate Th17 differentiation to favor induction of tolerogenic T regulatory cells (Tregs; [25, 56, 71, 82, 103, 107]). As Tregs critically regulate alloreactive T cell responses as well as induce transplant tolerance [27, 107], harnessing MSCs to induce both peripheral and central tolerance to allografts is an area of particular benefit to VCA applications.
B cell behavior, the other main cellular component of the adaptive immune system, is also altered in the presence of MSCs. Though less studied, MSCs have been shown to inhibit B cell proliferation in murine studies [18]. Specifically, allogeneic MSCs have been shown to inhibit the proliferation, activation, and immunoglobulin (IgG) secretion by B cells. IFNγ-induced IDO expression by MSCs has been linked to these effects as well [56].
Collectively, the powerful effects of MSCs on multiple pathways of the adaptive immune system (Fig. 17.2) are a promising approach to facilitating tolerance and eliminating the need for lifelong immunosuppression.
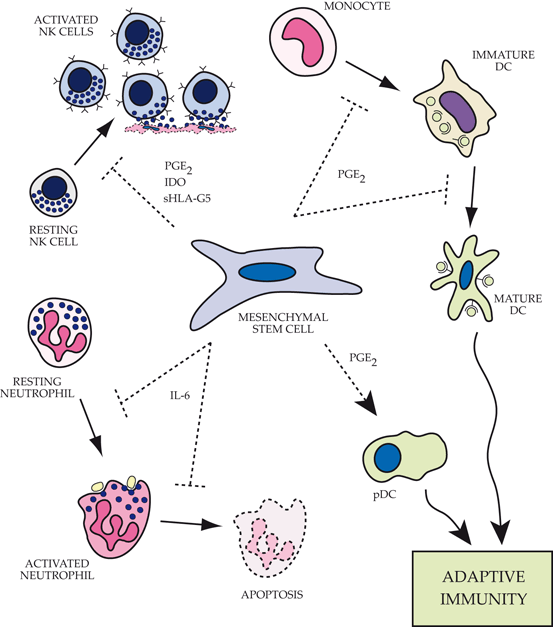
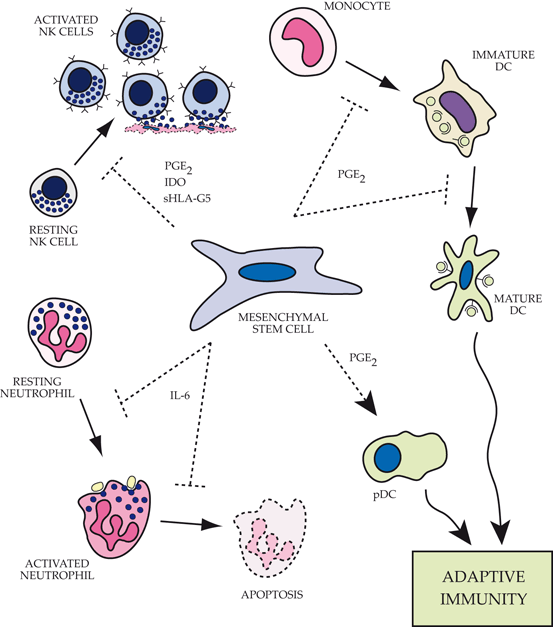
Fig. 17.2
Impact of mesenchymal stem cells (MSCs) on functions of the innate immune system.Top left: MSCs alter the phenotype of natural killer (NK) cells by inhibiting proliferation, cytokine expression and cell cytotoxicity via expression of a number of factors including prostaglandin E2 (PGE2), indoleamine 2,3-dioxygenase (IDO) and soluble HLA-G5. Top right: MSCs block the differentiation of monocytes into immature dendritic cells by preventing entry into the cell cycle. In addition, the maturation process of myeloid-derived dendritic cells is altered resulting in retention of more immature characteristics and an impaired ability to present antigen and activate the adaptive immune response. Bottom right: Plasmacytoid dendritic cells (pDC) significantly increase IL-10 expression in the presence of MSCs, promoting a more robust regulatory adaptive immune response. Bottom left: MSCs actively maintain neutrophils in a resting state, prevent apoptosis of both resting and activated cells, and decrease the respiratory burst associated with invading pathogens or inflammatory mediators via IL-6 secretion. PGE2 prostaglandin E2, IDO indoleamine 2,3 dioxygenase, sHLA-G5 secretory isoform of HLA-G, DC dendritic cells
The Innate Immune System, IRI, and MSCs
Traditionally, the focus of transplant immunology has been on targeting the mechanisms of adaptive immunity. However, there is an emerging consensus that the potency of rejection is strongly influenced by the activity of the innate immune system as well as external factors, particularly including IRI [51]. MSCs have an equally important role in modulating innate immune system response to allografts (Fig. 17.3).
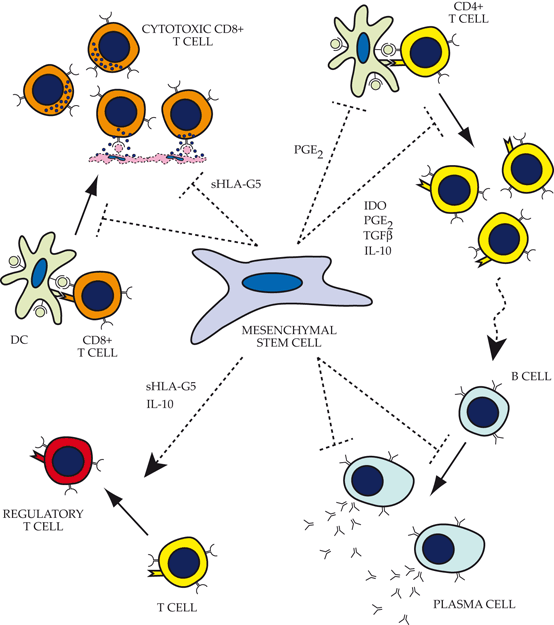
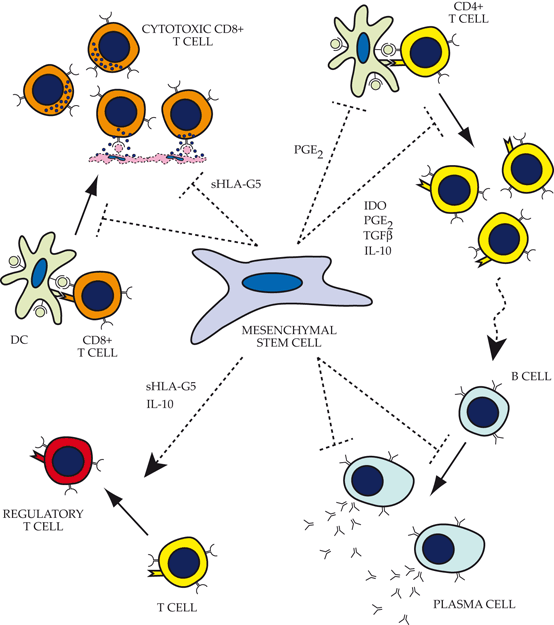
Fig. 17.3
Impact of mesenchymal stem cells (MSCs) on functions of the adaptive immune system. Top left: MSCs inhibit CD8+ T cell activation through a major histocompatibility complex -independent pathway (MHC-independent pathway). In addition, they also directly inhibit CD8+ cytotoxicity via expression of sHLA-G5. Top right: Several factors produced by MSCs actively contribute to direct inhibition of CD4+ activation, proliferation, and helper T cell function. Bottom right: MSCs inhibit B cell proliferation, differentiation, antibody production, and chemotaxis via its impact on helper T-cell function, as well as direct inhibition by MSC-expressed factors that are poorly understood. Bottom left: MSCs recruit and facilitate the expansion of regulatory T cells directly through expression of sHLA-G5 and indirectly through stimulation of IL-10 production by plasmacytoid dendritic cells
Natural Killer (NK) cells have a multifaceted role in allograft rejection. They contribute to the innate immune system’s ability to track pathogens through a surveillance role, and, within the lymph nodes, they produce significant amounts of IFNγ to activate T cell responses to potentiate allograft rejection [51, 58, 75]. NK cells cocultured with MSCs in the presence of IL-2 and IFNγ demonstrate reduced lytic capacity against traditional targets lacking MHC-1 expression [56]. In a manner analogous to its interaction with T cells, MSCs suppress not only natural killer (NK) cytotoxicity but also their ability to proliferate, largely through IL-6-, IDO-, and PGE-mediated mechanisms [103].
Like NKs, dendritic cells (DCs) are also members of the innate immune system. They are present mainly in tissues exposed to the external environment and serve as the most potent of the antigen-presenting cells (APCs) which are essential for immune system recognition of alloantigens. Both donor-derived and recipient-derived DCs have critical roles in triggering allograft rejection through direct and indirect pathways of allorecognition. MSCs interfere with the activation and maturation of DCs [1, 4, 21, 111] and tilt the immune response towards generation of tolerogenic phenotypes via IL-6-mediated mechanisms [34, 104]. Furthermore, MSCs downregulate expression of DC maturation markers including the major histocompatibility complex (MHC) class II, CD40, CD80, and CD86, and impair the ability of DCs to home to the lymph node in vivo [26]. DCs generated in the presence of MSCs produce high levels of anti-inflammatory cytokines including interleukin-10 (IL-10) and lower levels of TNFα. Functionally, tolerogenic DCs generated by MSCs have failed to induce activation of CD4+ T cells in vitro and in vivo [104]. The capacity of MSC-educated DCs to induce a state of peripheral tolerance would greatly improve outcomes in VCA, and early data have shown this mechanism to contribute to kidney allograft survival in the setting of low-dose immunosuppression [34].
Innate immune phagocytes including neutrophils and macrophages have been shown to promote graft rejection through tissue damage, production of pro-inflammatory cytokines, and activation of antigen-specific T cells [120]. Although it has not been directly shown in a transplant-related model, MSCs can reprogram macrophages to take on more “anti-inflammatory” (M2) phenotypes to mitigate propagation of injury to surrounding tissues [72, 74, 91, 98, 112]. Furthermore, MSCs actively maintain neutrophils in a resting state, prevent apoptosis, and decrease the respiratory burst associated with invading pathogens or inflammatory mediators via IL-6 secretion (Raffaghello 2008, p. 136).
IRI is an unavoidable consequence of VCA and has been associated with an increased incidence of both acute and chronic rejection in the solid organ literature [65]. Reactive oxygen species produced after reperfusion have been linked to the induction of adaptive immune responses through the activation of APCs. During reperfusion, allograft donor DCs are activated and recipient CD4+ T cells, monocytes, and macrophages infiltrate the reperfused graft. This results in a strong cytokine and chemokine release, including damage-associated molecular patterns (DAMPs; [24, 120]). Accordingly, there has been significant interest in using MSCs to mitigate this process. MSCs have been shown to protect against ischemia-reperfusion renal injury via inhibition of apoptosis and stimulation of cell proliferation [32]. Specifically, in the context of transplantation, MSC administration reduced allograft inflammatory gene expression and recruitment of APCs into the allograft in a model of renal allotransplantation [37]. Independent of their immunomodulatory activities, MSCs have been shown to release an array of growth factors to accelerate tissue repair including epidermal growth factor (EGF), fibroblast growth factor (FGF), platelet-derived growth factor (PDGF), transforming growth factor-β (TGF-β), vascular endothelial growth factor (VEGF), insulin-like growth factor 1 (IGF-1), angiopoietin-1, and stromal cell-derived factor-1 (SDF-1), all of which influence both stromal cells and endothelial cells [54, 55].
Taken together, there is overwhelming evidence demonstrating that MSCs modulate the function of immune cells in vitro, particularly with regard to T cell and APC behavior. While there is limited in vivo evidence corroborating the molecular mechanisms of these observations, as more complex in vivo models are developed, we will gain a better understanding of how to develop clinically effective MSC-based therapies.
MSC Targeting Strategies in Transplantation
In contrast to the multitude of studies examining the immunomodulatory properties of MSCs, there are relatively few studies examining therapeutic targeting strategies specifically in transplantation . Within transplantation, most models focus on MSCs in systemically delivered stem-cell transplants, with few studies focusing on solid organ (heart, liver, kidney), and even fewer on VCA [60, 62]. Analogous to solid organ transplantation, accelerated arteriosclerosis and vascular injury are hallmarks of chronic composite allograft rejection [99]. Both acute rejection and chronic rejection are characterized by significant vascular damage and accumulation of inflammatory cells within the allograft. Accordingly, strategies to utilize MSC-based cytotherapy should focus on protection of allograft’s endothelial barrier from injury. As MSCs have been shown to take residence in perivascular space [6, 8], they serve as ideal candidates for promotion of the endothelial barrier properties that are necessary to limit cytotoxic damage and influx of immune cells that prime the rejection response .
As with many therapies in transplantation, obtaining targeted delivery is a limiting factor in clinical efficacy. Generally, two approaches of systemic administration have been used for MSC applications. One is intravascular injection, utilizing the capabilities of MSCs to migrate to specific inflammatory tissues in vivo. The engraftment was demonstrated in animal models and capable of persisting as long as 13 months after transplantation [70]. However, studies observing MSC trafficking after systemic intravenous infusion have demonstrated that they largely end up accumulating in the lung, liver, and spleen rather than solely the site of injury [41, 42, 125, 36]. The other is site-directed delivery, such as direct injection [38], which can be impractical in the case of a composite allograft where there are multiple tissue compartments. In rodent transplant models [119, 124], MSCs effectively migrate to the site of allograft rejection during chronic rejection, suggesting that the inflammatory milieu of rejection can improve targeting and engraftment of MSCs. Pre-transplant infusion of MSCs appeared to be more effective as compared with peri-transplant administration. A recurring theme in these studies is that long-term graft acceptance is correlated to MSC-dependent expansion of Tregs or tolerogenic DCs in sites of immunologic importance. However, techniques to effectively traffic exogenous MSCs to those sites remains elusive [12, 13, 45, 88] and will remain a significant obstacle to clinical use of MSC immunotherapy .
As accelerated arteriosclerosis and vascular injury are hallmarks of solid/composite allograft rejection [99], strategies to utilize MSC therapy should focus on maintenance of the endothelial barrier from both ischemic and immunologic injury. As MSCs have been shown to take residence in perivascular space [6, 8], they serve as ideal candidates for promotion of the endothelial barrier properties that are necessary to limit cytotoxic damage and influx of immune cells that prime the rejection response. Novel strategies to improve MSC targeting have been discussed in the literature [17, 23, 35, 108, 114, 122] including hypoxic/pharmacologic preconditioning [122], genetic engineering of MSCs [17], and magnetic-based guidance [23, 35, 108, 114] to focus delivery to the region of interest using systemic delivery after the inciting inflammatory event. Recent work has proposed a new approach that can utilize MSC-based cell therapy to modify the allograft itself at the time of transplantation to facilitate graft tolerance [16, 76, 78, 100, 101] (Fig. 17.4). Ex vivo MSC perfusion utilizes clinically available organ perfusion technology [113] to engineer allografts that are primed for peripheral tolerance . MSCs are seeded into the allograft during the ex vivo period between procurement and transplantation where they take up permanent residence in the perivascular space of the allograft. Machine perfusion allows tight biologic control of the allograft environment in an isolated circuit and is clinically available for use in kidney, lung, and liver transplantation. The efficacy of pulsatile perfusion has been tested over the past 30 years, demonstrating a reduction in delayed graft function and improved long-term graft survival [16, 113]. The clear benefits of using ex vivo perfusion approach include the ability to target delivery of high numbers of immunosuppressive MSCs to the allograft using a clinically available technology that has been shown to reduce the effects of IRI injury and improve allograft survival .
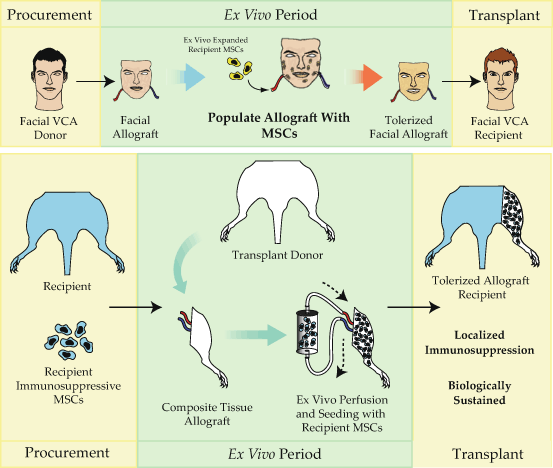
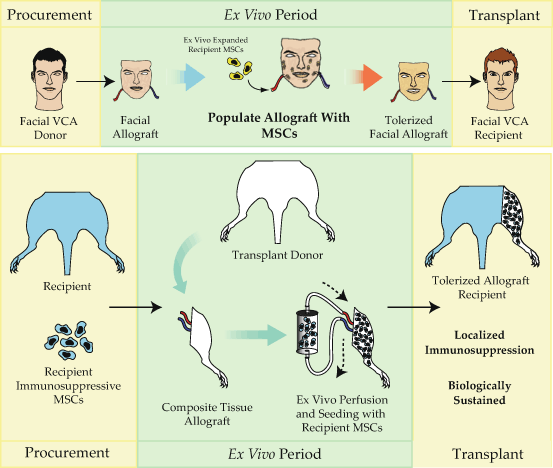
Fig. 17.4
The tolerization of composite tissue allografts. While MSCs are powerful immunomodulatory agents, effective targeting of these cells to exactly where they are needed remains a critical limitation of their use. Due to these limitations, we have recently developed a novel method of delivering immunosuppressive MSCs via the allograft vasculature during the obligate ex vivo period in between procurement and transplantation. Using this strategy, large numbers of immunomodulatory MSCs home to the perivascular niche within the allograft where they take up residence to intercept infiltrating leukocytes, potentially converting these effector cells to suppressive leukocytes, tolerogenic dendritic cells, and regulatory T cells
Applications of MSCs in Solid-Organ Transplant and VCA
There is a strong precedent set for use of MSCs in both preclinical and clinical settings to treat a variety of diseases and prevent acute rejection in transplantation. MSCs are an ideal cell-based therapeutic in many respects, given their impact on the innate and adaptive immune response . Their fundamental stem or progenitor cell properties make them a potentially self-renewing therapeutic agent which has the potential to persist for extended periods in the recipient. When delivered systemically, MSC preferentially home to known stem-cell niches, such as the bone marrow in a hand allograft, where they take up residence and modulate donor hematopoietic cells in this compartment. In addition to the bone marrow, MSCs have been demonstrated to migrate to ischemic or damaged tissues where they participate in both tissue regeneration and immunomodulation of the ensuing inflammatory response. Within the target tissue, they retain the capacity to migrate towards an ischemic or inflammatory stimulus, which may direct their movement through an allograft to areas undergoing acute rejection where they can exert their immunosuppressive effect. They also secrete a number of cytokines to attract subsets of leukocytes that egress from circulation during inflammatory processes, such as acute rejection, effectively attracting these effector cells to modulate their immune response. Finally, allogeneic MSCs evade the host immune system despite expressing intermediate levels of MHC class I on the cell surface [66, 86, 109], and fail to stimulate recipient lymphocyte proliferation [19, 67, 109]. This relative hypoimmunogenicity permits transplantation across allogeneic barriers, making third-party sources of MSCs a practical and viable alternative for use in VCA.
Since the first demonstration of prolongation of allograft survival in skin grafts over a decade ago [3], there have been numerous studies in small animal models to establish the powerful immunosuppressive role of systemically delivered MSCs in heart [12, 15, 22, 34, 45, 88, 124], islet cell (Ding 2009, p. 120; Li 2010, p. 122; Xu 2012, p. 124; Kim 2011, p. 128; Solari 2009, p. 130), kidneys (Ge 2010, p. 131; Casiraghi 2012, p. 87), and liver transplantation (Wang 2009, p. 30). While many of these experiments used no additional immunosuppression, more practical preclinical studies used low-dose immunosuppression that more accurately reflects the likely treatment strategy in human subjects. It remains to be determined, however, what the impact of the immunosuppressive drug regimen on MSC function and downstream generation of a regulatory T cell response will be (Zeiser 2006, p. 134; Wang 2009, p. 135).
There is an early yet growing clinical use of MSCs in solid-organ transplantation . In renal transplantation, a clinical feasibility study [83] demonstrated that autologous bone marrow-derived MSCs can be safely administered 7 days post transplant with concomitant increase in allograft Treg populations and stable serum creatinine at 1-year post transplant. Similarly, recent clinical trials in living-related kidney transplantation revealed that induction therapy with MSCs before allograft revascularization and then again 2 weeks following transplant resulted in lower incidence of acute rejection, decreased risk for opportunistic infections, and better estimated renal function after 1 year [106]. However, there is some evidence that post-transplant administration can induce a transient inflammatory allograft injury, not unlike “engraftment syndrome,” although there appeared to be no clinical consequences at 1-year posttransplant [83, 84]. Phase I studies are currently being conducted in liver transplantation [89] as well as lung transplantation [94].
Probably the most impressive in vivo evidence and use of MSC-based immunomodulation in humans is in the treatment of GvHD after allogeneic hematopoietic stem-cell transplantation. In phase II clinical studies, MSC infusions were shown to be safe and effective in treating steroid-resistant acute GvHD [68] and have shown to reduce the incidence of severe GvHD when used prophylactically [5, 64]. While extremely promising, further long-term studies are needed to conclusively demonstrate safety and efficacy.
Much like solid-organ transplantation, vascularized composite allotransplantation requires systemic lifelong immunosuppression. However, unlike the solid-organ transplant population, the recipients of reconstructive composite allografts are relatively healthy patients without evidence of end-organ failure or chronic debilitating disease. Considering the potential detrimental effects of systemic lifelong immunosuppression including shortened life span, organ failure, cancer, and even death, the need for more innovative therapeutic approaches towards achieving tolerance without the need for such harsh immunosuppressive drugs is even more evident in VCA.
There are important intrinsic differences in the composite allograft biological environment compared to solid organs that may represent an opportunity to use MSCs as potent immunomodulators. Unlike many solid organs, composite allografts frequently contain skin, fat, lymph nodes, and bone marrow, which are known stem-cell niches where MSCs may engraft and proliferate to exert their immunosuppressive effect. Thus, the allograft itself becomes a reservoir of immunomodulatory MSCs exactly where they are needed to exert their effect. The skin-containing allografts are a rich source of dendritic-antigen-presenting cells which frequently occupy similar tissue compartments that MSCs would engraft, potentially increasing the likelihood of generating large numbers of tolerogenic cell types. Furthermore, the very nature of a skin-bearing allograft being visible to the patient allows for continuous monitoring for signs of rejection, which would likely be a benefit when undertaking clinical trials of MSC therapy or minimization protocols. While there are no active clinical trials using exclusively MSCs for VCA, several preclinical studies have demonstrated a marked immunosuppressive effect of systemically delivered MSCs following VCA on the incidence and severity of acute rejection, the peripheral blood T regulatory response, inflammatory cytokine expression profile, and ultimately allograft survival [2, 59, 61, 63]. These promising findings serve as a foundation for eventual translation of MSC therapy to clinical cases.
Future Directions and Considerations
As promising as MSCs appear as therapeutic agents, some consideration must be made to their safety profile. One of the current disadvantages of systemic immunosuppression regimens is their inability to distinguish between pathologic and protective immune responses; it is important to critically examine the effects of MSC-based immunomodulation. There is little existing knowledge regarding the in vivo survival of MSCs, their potential to contribute to systemic immune suppression, ectopic tissue formation, or malignancy [14
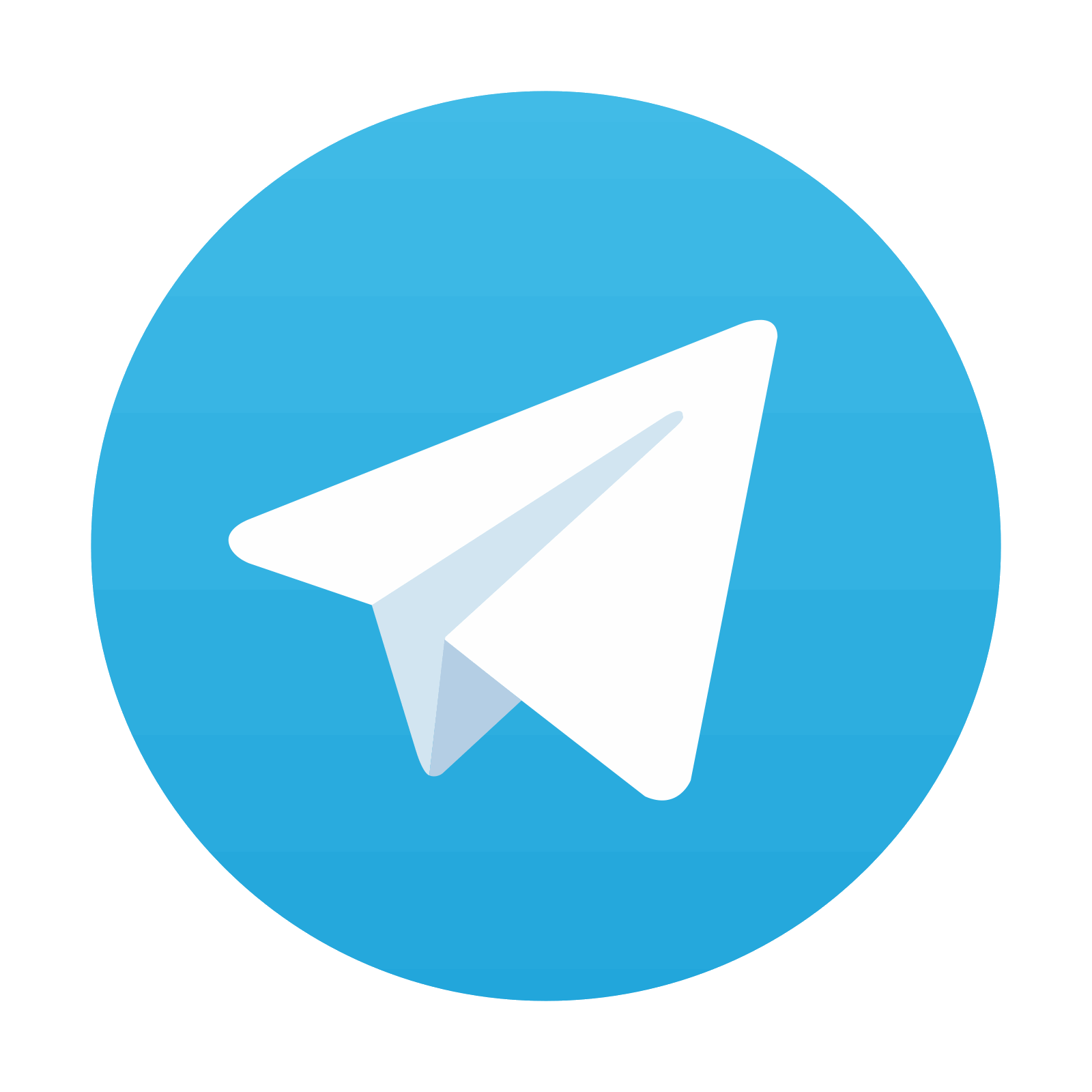
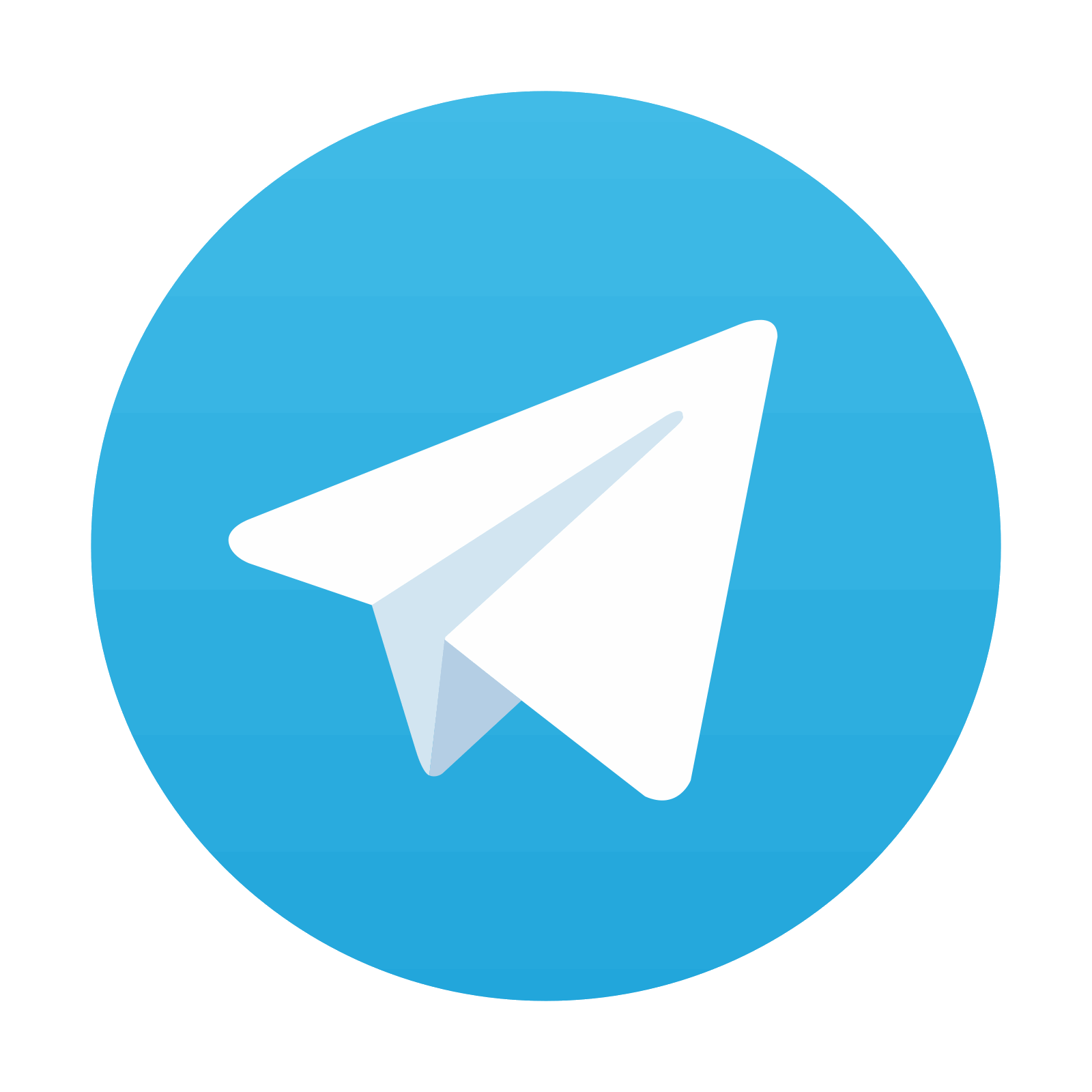
Stay updated, free articles. Join our Telegram channel
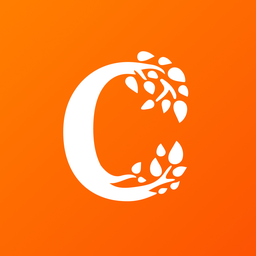
Full access? Get Clinical Tree
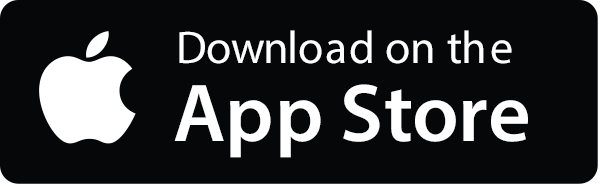
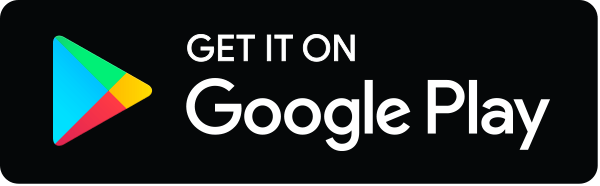