The neural activity responsible for voluntary facial expression relies on an intricate set of interconnections distributed across multiple cortical facial motor areas, the amygdala, and other subcortical areas. The target end-organs for this intricate neural system are the facial muscles directly innervated by last order postsynaptic axons in the facial nerve. The muscles of the upper and lower face are controlled by anatomically distinct motor areas reliant on distinct patterns of neural activity. Disruption of this intricate neural network, when followed by spontaneous recovery or surgical repair, is commonly associated with abnormal facial movements such as synkinesis. Synkinesis is the undesired synchronization of an involuntary muscle group triggered by a desired voluntary contraction of another muscle group. Synkinesis can occur in any functional muscle group in the body but is commonly seen after facial nerve injury. A typical example of facial synkinesis is seen when a voluntarily blink is accompanied by co-contraction of lower facial muscles around the lips (ocular-oral synkinesis) and when narrowing of the palpebral fissure occurs when smiling (oral-ocular synkinesis). In its mildest form, facial synkinesis is tolerable and often ignored by patients but can be very debilitating when severe.
The pathological mechanism of synkinesis is not completely elucidated and as a result, current treatment options have mixed efficacy. Presently, synkinesis treatment is targeted towards peripheral facial nerves and muscles in the form of chemodenervation, selective myectomy and selective neurectomy. To minimize the sequelae of synkinesis post facial nerve injury, and to offer more effective rehabilitative and therapeutic treatment, it is necessary to better understand the response of peripheral nerves to injury, the process of nerve regeneration, electrophysiologic changes in peripheral facial nerves post injury, and any associated cortical adaptations.
Etiology and Epidemiology
Faulty regeneration is the norm and not the exception following facial nerve injury of any cause. Facial synkinesis has been reported in a wide range of ages from infants to elderly patients and does not appear to be limited to a specific demographic. The most common causes of facial paralysis, such as Bell’s palsy, Ramsey Hunt syndrome, trauma, and postsurgical injury, have all been associated with synkinesis to varying degrees. In one study, synkinesis was clinically observed in 55% of patients. Using electrophysiologic criteria, synkinetic movement can be detected in nearly all patients with proximal facial nerve injury. In one of the largest studies evaluating the natural course of idiopathic peripheral facial nerve palsy, synkinesis was recorded in about 16% of patients. In a retrospective study of 102 patients with untreated Ramsay Hunt syndrome, all patients who initially developed complete paralysis also developed synkinesis, whereas synkinesis was recorded in 10% to 15% of those with incomplete paralysis. Pregnancy, advanced age, and diabetes have been studied as potential risk factors for Bell’s palsy but have not shown to be linked with severity of synkinesis. However, there is a clear relationship between the severity of facial palsy and the degree of synkinesis. This link is further supported by electrophysiologic studies showing that patients with a prognostic electroneurography (ENoG) value of less than 10% have higher risks of aberrant regeneration and moderate-to-severe synkinesis than those with an ENoG value of 10% to 20%.
The Facial Neuromuscular Network
The facial nerve is a mixed motor and sensory nerve composed of approximately 10,000 neurons. About 7000 of these neurons are motor and innervate the muscles of facial expression. The motor fibers originate from the motor face area of the frontal lobe and project via the corticobulbar tract and the internal capsule to the facial nucleus. These afferent motor fibers synapse with nuclei within the facial nucleus arranged into four major subgroups: dorsomedial, ventromedial, intermediate, and lateral. Each nuclei subgroup projects to a specific group of facial muscles and explains, in part, the intricate voluntary control of facial muscle movement. The degree of nuclei representation and projection to muscle groups are related to the functional and behavioral importance of each muscle. For example, the subnuclei representing the orbicularis oris and oculi are prominently represented compared to those of less important muscles such as the depressor septi nasi or auricularis muscles. Much of what is known about this facial nucleus sub-innervation in humans is extrapolated from tracer injection studies in primates. , The corticobulbar projections were defined by injecting anterograde tracers into the face representation of each motor cortex, and the musculotopic organization of the facial nucleus was defined by injecting fluorescent retrograde tracers into individual muscles of the upper and lower face. Following these injections, the facial nucleus was noted to received input from all face representations. Injections in all cortical face representations labeled terminals in all nuclear subdivisions (dorsal, intermediate, medial, and lateral). However, significant differences occurred in the proportion of labeled boutons found within each functionally characterized subdivision.
Once the facial nerve exits the brain stem, the discrete compartmentalization of motor neurons destined for specific muscle groups is lost. Consequently, the fibers to all facial muscles are distributed throughout the nerve at all levels before peripheral branching occurs in the parotid. Within the parotid gland, the facial nerve divides into a superior (temporofacial) and an inferior (cervicofacial) division, interconnected but containing fibers destined to specific muscle groups. The delicate musculotopic arrangement of the facial nucleus and facial nerve is disrupted following facial nerve injury and explains in part abnormal motor recovery.
When A Nerve Is Injured
The axons that make up a motor nerve are myelinated and arranged in a fascicular architecture that is both protective and suited for rapid nerve conduction. The myelin sheath is made and maintained by Schwann cell membranes wrapped compactly around the axon. Myelin is in turn coated by a basal lamina, outside of which lies the endoneurium, which contains fibroblasts, blood vessels, and a few macrophages, which is ultimately surrounded by a multilayered cellular tube, the perineurium. This assembly of axons, Schwann cells, and connective tissue makes up a nerve. Injury to the facial nerve may disrupt the nerve architecture to varying degrees depending on the extent of insult. Seddon and Sunderland classified the fundamental types of nerve injury based on the extent of disruption of the endoneurium and perineum as well as the integrity of the axon. The imperfect response of peripheral nerves to injury underlies the clinical manifestation of synkinesis.
In response to injury, both neurons and Schwann cells switch to a cell state suited for nerve repair and regeneration. In damaged neurons, the signal switch to repair mode is heralded by the activation of an extensive gene program that facilitates axonal regeneration, a response classically referred to as the cell body response. Similarly, Schwann cells change into a phenotype primed for repair to facilitate nerve regeneration. This transformation to a repair-sustaining Schwann cell phenotype is mediated by a complex cellular process that is time dependent. Early after nerve injury, the Schwann cells distal to the damaged area lose contact with axons as they degenerate. The loss of contact is by itself a trigger for Schwann cell transformation. Invading macrophages secrete bioactive factors which, together with the loss of axonal contact, influence the change in surrounding Schwann cells toward a repair type cell.
Within their original basal lamina tubes, these transformed repair type Schwann cells aid in clearing degenerated myelin by autophagy. The released cytokines call in macrophages for further myelin clearance. The Schwann cells elongate forming regeneration tracts, Bunger bands, which guide regenerating axons towards their target. Axons that successfully grow across the regeneration zone induce transformation of the repair Schwann cells back to the myelin phenotype and become ensheathed in a new myelin membrane. This remarkable, adaptive injury response of neurons and Schwann cells is clinically inadequate in severe and long-lasting injuries. There are several reasons for this inadequacy. , First, the early regeneration-enabling environment that enables nerve regeneration is unstable as the number Schwann cell dwindles. The number of cells that can be isolated from nerves after 6 months of chronic denervation is only 10% to 15% of that obtained at 4 weeks. Second, with prolonged denervation, the remaining Schwann cells become less effective with surviving cells gradually down-regulating their repair phenotype, followed by the death of cells that have by that time lost most of their regeneration-supportive properties. Clinically suboptimal reinnervation of the facial muscles manifest as hypotonus, hypertonia, myokymia, or synkinesis.
Proposed Mechanisms for Synkinesis
Three main theories—aberrant regeneration of facial nerve fibers, ephaptic transmission of impulses, and somatotopic reorganization of the facial nucleus—have been forwarded as potential mechanisms for synkinesis. More recently, there has been increasing understanding of the potential role played by electrophysiologic changes in the peripheral nerve and cortical adaptations in postinjury synkinesis.
Aberrant Regeneration
Injuries that disrupt the endoneurium can result in interrupted and misaligned regeneration tracts. In addition, mechanical barriers at the injury segment compounded by the slow growth of proximal axonal stumps limits the number of axons that successfully cross, resulting in misrouting and reinnervation errors. Misrouted axons meant for the orbicularis oris muscle end up in the orbicularis oculi muscle and result in ocular-oral synkinesis. Recent studies have suggested that the opportunity for misdirection of axons does not only occur at the injury site, but that aberrant axonal growth occurs throughout the length of the nerve. The most commonly observed clinical patterns of synkinesis are predictable rather than random, with mouth and eyelid coupling the most common occurrence. The mechanism of misrouted axons is the most commonly stated reason for synkinesis and underlies the treatment method of selective neurectomy, chemodenervation, and myectomy.
Peripheral Ephaptic Transmission
The ability of Schwann cells to restore myelin sheath in extensively degenerated nerve is imperfect and incomplete. Consequently, regenerated axons with defective myelin membranes can result in “short circuiting” with cross-excitation of adjacent axons meant for different target muscles. Ephaptic coupling of transmission between adjacent axons is distinct from impulse spread across synapsis. Rather it is the spreading of impulses along and across adjacent axons such that action potential propagating along one axon fires up an adjacent axon. The term ephaptic was coined from the Greek word “ephapse” signifying the act of touching (as opposed to “synapse” or linking) by Arvanitaki in a study of nerve conduction in giant axons of Sepia officinalis (common cuttlefish). Since then, the term ephaptic interaction has been used to refer to communication between neuronal cells via electrical conduction through the surrounding extracellular space, as opposed to communication mediated by chemical synapses or gap junctions. Ephaptic transmission is usually inhibited by myelination but can occur when remyelination is incomplete. The coexcitation of different muscle groups will, as a result of ephaptic impulse transmission, result in synkinesis. The concept of ephaptic coupling of adjacent axons was investigated by Katz and Schmitt in 1940, who showed nonsynaptic electrical interaction between adjacent nerve fibers. In their work, two large parallel nonmyelinated axons were isolated from the crab limb nerve. They demonstrated that: (1) the passage of an impulse in one fiber causes subthreshold excitability changes in the adjacent fiber and (2) when impulses are set up simultaneously along both fibers, a mutual interaction occurs that can lead to speeding up or slowing down of the impulses and also possibly to synchronization between the two impulses, depending on the initial phase relationship. In 1984, ephaptic interactions were suggested to play a role in hemifacial spasm pathophysiology by causing “cross-talk” between facial nerve fibers.
Nuclear Hyperexcitability
Enhanced excitability of the facial nucleus has also been suggested as a possible mechanism for synkinesis following facial nerve injury. This is supported by the predictable and nonrandom pattern of synkinesis and the clinical observation of synkinesis early in the postinjury period within a time line that is neither explained by aberrant nerve regeneration or ephaptic impulse transmission given that axons grow at a rate of only 1 mm/day. Nuclear hyperexcitability is believed to occur when inhibitory signals to the facial nucleus are lost in response to peripheral facial nerve injury. Stripping of synaptic input into the facial nucleus has been observed within days of facial nerve injury. The time line for this synaptic stripping matches the observation of synkinesis as early as 4 to 8 weeks following the onset of idiopathic facial palsy. ,
The first evidence for disconnection of synapses following peripheral nerve injury in humans was reported in an autopsy study of a 77-year-old man who died from an unrelated cause 3 months after developing left facial paresis from otitis media. Microscopic evaluation and immunohistochemistry revealed a striking reduction in the number of afferent synaptic contacts and marked chromatolytic changes in the left facial nucleus. Immunocytochemistry for synaptophysin revealed a marked loss of afferent synaptic contacts from somatic and stem dendritic surface membranes of all chromatolytic motor neurons. Wrapping of a number of neurons by newly formed glial fibrillary acidic protein-positive astrocytic cell processes could be detected in the regenerating facial motor nucleus. These changes were absent from the contralateral, normal-appearing facial nucleus. The marked occurrence of synaptic stripping, preferentially of inhibitory nerve, can explain enhanced nuclear hyperexcitability and poorly controlled bursts of undesired muscle contraction.
Axonal Hyperexcitability
Fundamental to nerve conduction is the maintenance of a resting membrane potential and rapid impulse propagation due to the exchange of sodium (Na + ) and potassium (K + ) across ion channels in response to depolarizing and hyperpolarizing stimuli. The arrangement of myelin around nerves, with myelin free segments at the node of Ranvier and the electrophysiologic characteristics of ion channel distribution at the nodal and internodal segments, facilitates the speed of impulse conduction. Traditionally, nerve conduction studies that measure impulse conduction velocity, latency, amplitude, and F-waves have been used clinically to assess the gross status of peripheral nerves. More recently, axonal excitability study has emerged as a noninvasive technique that offers complementary information to that provided by conventional nerve conduction studies, to gain more insight into axonal function with detailed information about the axonal membrane potential and ion channel properties. The key axonal excitability parameters commonly measured after delivery of impulse to a test nerve include threshold, strength-duration time constant, rheobase, threshold electrotonus, recovery cycle, and current/threshold relationship. Changes in these axonal biophysical parameters have been attributed to aberrant ionic conductance, particularly pertaining to Na + and K + exchange, and has been characterized for a diverse range of conditions including toxic, metabolic, both acquired and inherited demyelinating neuropathies, and neurodegenerative disorders such as amyotrophic lateral sclerosis (ALS), as well as providing insight into pathophysiologic changes occurring at the peripheral nerve level in disorders of the central nervous system such as stroke, spinal cord injury, and multiple sclerosis. , Using axonal excitability techniques, recent studies have explored the possible contribution of electrophysiologic changes in peripheral facial nerves following injury that may predispose to ectopic activity such as spasms and synkinesis. In one study, facial nerve excitability parameters were compared between patients with synkinesis and those without following facial paralysis. Synkinesis patients demonstrated marked alterations in a number of excitability parameters compared with normal controls. Specifically, there was depolarized resting membrane potential in the synkinesis group predisposing to axonal hyperexcitability hyperkinesis and myokymia. As in ALS, the alteration in resting membrane potential of injured facial nerves was attributed to changes in the distribution of Na + channels in the injured nerves. Acute Na + channel dysfunction has been directly implicated in the pathophysiology of virus-induced facial palsy and has been linked to the hyperexcitability observed in postinjury facial nerve. , In one study, the neurotropic virus implicated in Bell’s palsy, herpes simplex type 1 (HSV-1), was found to inhibit the excitability of the facial nerve by an elective, precipitous, and complete internalization of voltage-activated sodium channel proteins from the plasma membrane of the neurons. This change in the transmembrane sodium channel was RNA-mediated and occurred directly at the axonal site of virus transfection. This ability of an axon to modify protein transcription at the site of the viral exposure or injury has emerged as a key mechanism in the response of peripheral nerves to injury. Axonal excitability studies in Bell’s palsy patients shows a pattern that lends further support to the role of Na + channels in the sequelae of facial nerve injury. This evolving understanding of the pathophysiology of peripheral facial nerve injury offers opportunities for preventative and therapeutic intervention. For example, medications that stabilize membrane potential through their effect on Na + channels may be considered early in the recovery of idiopathic facial nerve paralysis even before synkinesis becomes clinically evident. Additionally, excitability parameters may be used to select appropriate nerves for reanimation procedures or selective neurectomy.
Cortical Adaptation
The fine voluntary control of facial muscles begins in the motor cortex. The previously described mechanisms for synkinesis all pertain to the facial nerve from the nucleus in the pons to the extracranial nerve. Given the known ability of the brain to undergo plasticity, the role of cortical changes following facial nerve injury as a potential cause of defective motor control is being investigated. The development of sophisticated noninvasive neuroimaging techniques over the past decade has made it possible to study cortical changes in response to peripheral nerve injury. In a study of patients with facial synkinesis, functional magnetic resonance imaging (fMRI) demonstrated that the distance between motor cortical subsites activated on ipsilateral blinking and smiling tasks was decreased on the affected half of the face compared with the unaffected side. In another study investigating resting state fMRI parameters in post-facial paralysis patients, there was an abnormal state of hypercompensation in motor control areas in patients with compared with patients without facial synkinesis. , Together, these studies support the role of a functionally aberrant motor control system in facial synkinesis. Facial retraining therapy with biofeedback is an integral aspect of the management of facial synkinesis. Recent reports indicate that people are able to develop control over the activity of specific brain areas when provided with real time–fMRI biofeedback. , The use of real time–fMRI biofeedback in the treatment of facial synkinesis is an interesting research tool that may hold therapeutic potential.
Summary
Faulty facial nerve regeneration manifesting as synkinesis is a challenging sequelae of facial nerve injury for both clinicians and patients. The varied pathophysiologic mechanisms of synkinesis, encompassing misdirected axons, ephaptic coupling, synaptic stripping with neuronal hyperexcitability, axonal excitability from altered ion channel function, and cortical adaptation, underscores the difficulty in establishing a universally effective preventative and therapeutic intervention. However, improved understanding of the electrophysiologic alterations that accompany peripheral nerve injury and new clinical tools available for characterizing the excitability parameters of individual nerve branches offer a selective and targeted rebalancing of the disorganized facial neuromuscular circuit using cortical retraining therapy, effective pharmacotherapeutics, selective myectomy, and neurectomy.
References
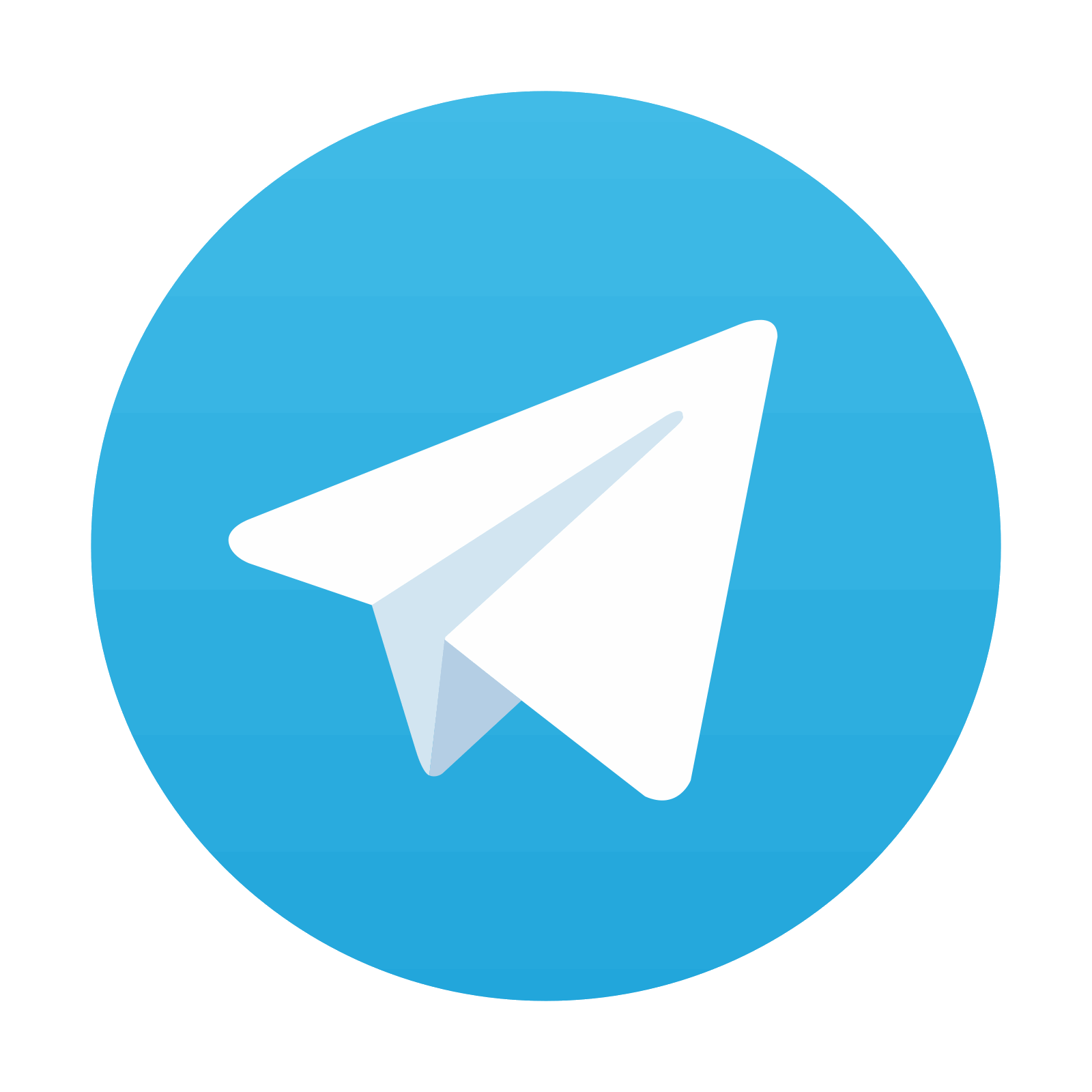
Stay updated, free articles. Join our Telegram channel
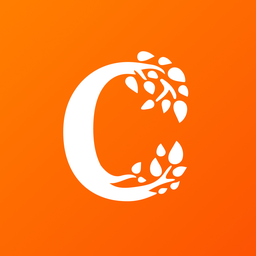
Full access? Get Clinical Tree
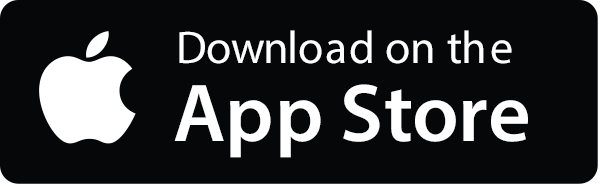
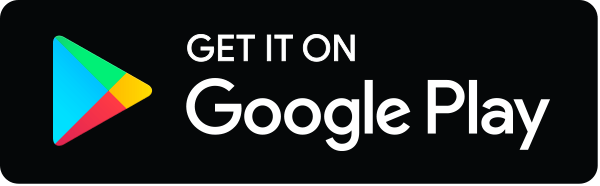
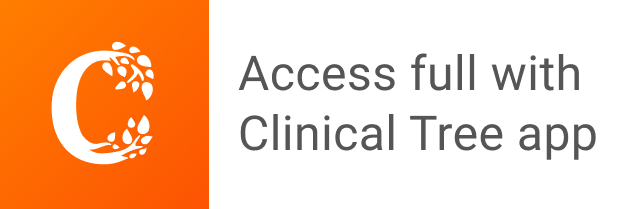