Fig. 15.1
(a) Pinhole in front of the detector rejects light emerging from out of focus regions. (b) Schematic representation of CLSM optics (Semwogerere and Weeks 2008)
Schematic representation of a CLSM is provided in Fig. 15.1b. In a modern CLSM, a beam of light is provided by a laser which passes through a pinhole onto a dichroic mirror which directs the light to a scanning mirror assembly and onto the specimen through the microscope objective. The scanning mirrors scan the laser across the specimen in order to generate the image. Light reflected from the specimen is de-scanned at the scanning mirrors and passes to the photo-multiplier tube (PMT) detectors through the dichroic mirror (Semwogerere and Weeks 2008). Light coming from out of focus regions of the specimen is blocked at the detector by a pinhole. The image of the specimen is reconstructed by a computer from the spatial coordinates and corresponding light intensity at the detector.
15.2.2 Major Advantages of CLSM
Blurring effect observed in the conventional microscope is either eliminated or reduced to a great extent in CLSM, and as a result, images generated are very sharp, have a high contrast and a higher resolution. In practice a CLSM can have at best a horizontal resolution of 0.2 μm and at best a vertical resolution of 0.5 μm. Further, a 3D sectioning or optical sectioning of the sample is possible as a very small focal point is imaged at a time which also gives possibility to image thicker specimens using a confocal microscope. Using multiple lasers, it is possible to excite different fluorophores simultaneously.
15.2.3 Major Disadvantages of CLSM
CLSM has an inherent resolution limitation of about 0.2 μm and is dependent on the wavelength of excitation. Due to the high intensity light source, photo bleaching and photo-damage in the illuminated region of the specimen is possible and repeated scans with high-energy light beam greatly reduce the viability of biological tissues and thereby the available time for studying a given specimen. For a high quality image, CLSM usually needs long scan times and as a result, CLSM is not suitable for imaging rapid physiological events in a cell or a tissue. In addition, because lasers emit light only at certain narrow bandwidths, in order to be able to excite a large range of fluorophores, multiple lasers need to be employed which along with the complex electronics, scanning mirrors, and sophisticated data acquisition system raise the cost.
15.2.4 CLSM Used for Tracking Liposomal Formulations in the Skin
Liposomes have been extensively studied and suggested as a vehicle for topical drug delivery (Chen et al. 2010, 2011; Dragicevic-Curic et al. 2008, 2009; Ntimenou et al. 2012). However, the mechanism of penetration of liposomes as drug carriers into the intact skin is not fully understood. In this section, we will discuss the interactions between liposomes, containing hydrophilic and lipophilic fluorescent probes, and human as well as rat skin using CLSM.
15.2.4.1 Tracking Skin Penetration of Liposomally Entrapped or Un-entrapped Hydrophilic Fluorescent Model Drug
The mechanism of action of liposomes as penetrable drug carriers in topical delivery is not completely understood. In order to evaluate if liposomes are able to increase skin penetration of only entrapped hydrophilic drugs or also of unentrapped hydrophilic drugs, three different liposomal formulations using carboxyfluorescein (CF) as a fluorescent hydrophilic model drug were prepared: ‘CFin-out’ (non-entrapped CF was not removed), ‘CFin’ (non-entrapped CF was removed), and ‘CFout’ (a pre-calculated amount of CF was added to empty liposomes). All the liposomal formulations had a similar size of liposomes and the concentration of CF was the same in all formulations (Verma et al. 2003b). After 6 h incubation of the skin with different formulations, the skin was cryofixed and 7 μm thick sections were cut using a cryo-microtome. These cross-sections were investigated using CLSM for the skin penetration of CF.
The penetration studies and CLSM images showed that liposomes CFin-out exhibited maximum deposition of CF in the SC, whereas liposomes CFin showed higher penetration of CF into the deeper skin layers such as the viable epidermis (Fig. 15.2), and through the skin to the acceptor compartment of the Franz diffusion cell. These results were comparable to the data obtained from tape stripping experiments. This study confirmed the assumption that liposomes CFin-out were not under osmotic stress and will, therefore, transfer themselves more easily into the SC. The results indicated further that phospholipid vesicles not only carry the entrapped hydrophilic substance, but also the non-encapsulated hydrophilic substance into the SC and possibly to the deeper layers of the skin. Although CLSM served as a useful tool to estimate skin penetration, CLSM images do not provide the visualization of single liposomes, so the exact mechanism of penetration of liposomes still remains an unsolved question.


Fig. 15.2
Fluorescence micrographs of cross-sections of human abdominal skin incubated on Franz diffusion cells with different formulations containing CF. Formulations were applied without occlusion for 6 h. (a) Liposomes CFin; (b) liposomes CFin-out; (c) liposomes CFout, CF outside liposomes; and (d) CF dissolved in Tris buffer. Scale bar represents 100 μm (Reprinted from Verma et al. (2003b) with permission from Elsevier)
15.2.4.2 Effect of Vesicle Diameter on Skin Penetration of Liposomally Entrapped Drugs
The influence of vesicle size on the penetration of fluorescently labeled liposomes into the human skin was investigated using lipophilic fluorescent label, 1,1′-dioctadecyl-3,3,3′,3′-tetramethylindocarbocyanine perchlorate (DiI) (Verma et al. 2003a). In all CLSM images (Fig. 15.3) very high fluorescence was observed in the SC, which was expected due to the lipophilic nature of the fluorescent label, DiI. This study indicated that large vesicles with a size ≥ 600 nm were not able to deliver their contents into the deeper layers of the skin. These liposomes probably stayed in/on the SC and after drying they formed a layer of lipid, which would have further strengthened the barrier properties of the SC. The liposomes with size ≤ 300 nm were able to deliver their contents to some extent into the deeper layers of the skin. However, the liposomes with size ≤ 70 nm were most promising for dermal drug delivery into the deeper skin layers as they showed maximum fluorescence both in viable epidermis, as well as in dermis.


Fig. 15.3
Fluorescence micrographs of cross-sections of human abdominal skin incubated on Franz diffusion cells with different formulations containing DiI. The vesicles were applied non-occlusively for 12 h. (a) Ethanolic solution of DiI; (b) NAT8539/ethanol (10/3.3); (c) NAT8539/ethanol (10/10); (d) NAT8539/ethanol (10/20). Scale bar represents 50 μm (Reprinted from Verma et al. (2003a) with permission from Elsevier)
15.2.4.3 Synergistic Penetration Enhancement Effect of Ethanol and Phospholipids on Topical Drug Delivery
It was observed that the composition of liposomal formulation had an appreciable effect on the penetration of compounds into and through the skin (Hofland et al. 1994; Jimbo et al. 1983; Loftsson et al. 1989; Tenjarla et al. 1999). Hence, the effect of lipid vesicular systems embodying ethanol in relatively high concentrations on the percutaneous penetration of cyclosporin A (CyA) was investigated using a standardized skin stripping technique and CLSM (Verma and Fahr 2004). Ethanol has been widely reported as an efficient skin penetration enhancer in the concentration of 5–100 % (Bhatia and Singh 1999; Kobayashi et al. 1994; Simonetti et al. 1995). In a preliminary study, we have seen that not only are the amount and the type of phospholipids important for skin penetration enhancement, but also the amount of ethanol has a significant role in delivering the fluorescent model compounds into the skin (Fig. 15.4) (Verma 2002). We hypothesized that ethanol and phospholipids might have synergistic skin penetration enhancing effect.


Fig. 15.4
Fluorescence micrographs of cross-sections of human skin incubated with vesicles with different lipid content for 12 h. (a, b) Ethanolic solution of N-Rh-PE (a) and CF (b). (c, d) Flexible liposomes with N-Rh-PE (c) and CF (d). (e, f) PL 90H-liposomes with N-Rh-PE (e) and CF (f); (g, h) PL25 liposomes with N-Rh-PE (g) and CF (h)
In order to evaluate synergistic effect of the ethanol and phospholipids on penetration of DiI, vesicles with composition of 10 % (w/v) phospholipid mixture NAT 8539 (Lipoid, Germany) and ethanol at different concentrations ranging from 0 to 20 % w/v were prepared. The results of the CLSM studies are represented in Fig. 15.5. Ethanolic solution of the DiI dye was able to deliver only weak fluorescence into the SC, and no fluorescence was noticeable in the viable epidermis and dermis (Fig. 15.5a). Formulation, NAT 8539/ethanol (10/3.3), produced a homogeneous bright fluorescence throughout the SC, but no fluorescence was observed in the viable epidermis and dermis (Fig. 15.5b). Formulation, NAT 8539/ethanol (10/10), produced a bright fluorescence throughout the SC with very weak to weak fluorescence observed in the viable epidermis and dermis (Fig. 15.5c). Formulation NAT 8539/ethanol (10/20) produced a homogeneous bright fluorescence throughout the SC and very a weak fluorescence was noticeable in the viable epidermis and dermis (Fig. 15.5d). CLSM experiments have shown that the ethanolic solution of DiI was not even able to deliver the fluorescent label into the SC. In contrast, all the formulations with NAT 8539 and ethanol produced a bright fluorescence homogeneously throughout the SC. Formulations prepared with NAT 8539 containing 10 and 20 % ethanol were also able to show very weak fluorescence in the viable epidermis and dermis. The results above were confirmed by in vitro penetration studies followed by tape stripping and extraction of the radioactively labeled CyA from various layers of the skin. Ethanol, together with NAT 8539 had synergistic effects on the delivery of the CyA into the skin, and the enhancement effect of ethanol was concentration dependent. Although CLSM images do not provide pure quantitative data regarding the skin penetration, they are, however, extremely useful as a comparative tool and give useful information about the distribution of the drug within the skin.


Fig. 15.5
Fluorescence micrographs of cross-sections of human abdominal skin incubated on Franz diffusion cells with different formulations containing DiI. The vesicles were applied without occlusion for 12 h. (a) Ethanolic solution of DiI; (b) NAT8539/ethanol (10/3.3); (c) NAT8539/ethanol (10/10); (d) NAT8539/ethanol (10/20). Scale bar represents 50 μm (Reprinted from Verma and Fahr (2004) with permission from Elsevier)
15.2.4.4 Terpenes as Penetration Enhancers in Liposomes
In this study, CLSM was used to investigate if incorporation of penetration enhancers (terpenes) into liposomal formulations had an effect on their percutaneous penetration enhancing ability in human and rat skin (Verma 2002). Fluorescent derivative of CyA, D-Ala-8-CS-beta-aminebenzofurazan (Fl-CyA) and DiI, were used as lipophilic markers while Alexa Fluor-488® (Life Technologies GmbH, Germany) was used as a hydrophilic fluorescent marker. Vesicles with and without terpenes were compared with ethanolic and hydro-alcoholic solutions of the fluorescent labels. Double-labeled vesicles, vesicles containing both DiI and Alexa Fluor-488®, were applied to skin for 6 and 12 h. Penetration of the fluorescent labels was visualized by CLSM both in terms of depth and intensities of the fluorescence. Fluorescent intensities of the CLSM images were semi-quantitatively scored ranging from no fluorescence to bright fluorescence. Further details regarding imaging and formulations can be found elsewhere (Verma 2002).
CLSM images of cross-sections of rat skin incubated for 6 h with Fl-CyA liposomes prepared with and without terpenes are compared in Fig. 15.6. A bright fluorescence was observed in the SC (Fig. 15.6b) for skin treated with Fl-CyA liposomes without terpenes, but only a negligible or no fluorescence was observed in the viable epidermis and dermis. The skin treated with Fl-CyA liposomes containing 1 % terpenes showed a relatively higher fluorescence in the SC and weak fluorescence in the epidermis, suggesting diffusion of the Fl-CyA from SC to the epidermis (Fig. 15.6c). Ethanolic solution of Fl-CyA showed a very weak fluorescence in the SC and no fluorescence was observed in the viable epidermis and dermis (Fig. 15.6a). Based on the fluorescence scores obtained from CLSM images, results indicate that penetration enhancers do play an important role in the penetration of fluorescent labels into the skin.


Fig. 15.6
Cross-sections of rat skin incubated with different formulations containing Fl-CyA. The vesicles were applied non-occlusively for 6 h on rat skin. (a) Ethanolic solution of Fl-CyA, (b) Fl-CyA liposomes without terpenes, and (c) liposomes containing 1 % terpenes. The length bar represents 10 μm. Arrows represent hair follicles in the dermis
Cross-sections of human skin treated with double-labeled (DiI and Alexa Fluor 488®) liposomes with and without terpenes are represented in Fig. 15.7. Incubation period of formulation with the skin was 6 h (Fig. 15.7a–c) and 12 h (Fig. 15.7d–f). Control was provided by skin samples treated with hydro-alcoholic solution containing both dyes mentioned above. In all the samples, the fluorescence was restricted mainly to the SC and to a smaller or larger extent, to the viable epidermis. Samples treated with hydro-alcoholic solutions of the dyes exhibited fluorescence for both DiI and Alexa Fluor-488® only in the SC. Increasing the incubation period from 6 h to 12 h only increased the fluorescence intensities for both the labels (Fig. 15.7a, d). In samples treated with dual labeled liposomes without terpenes, fluorescent markers after 6 h diffusion are restricted to the SC only. DiI penetrated up to deeper layers of the SC however no fluorescence was observed in the viable epidermis and dermis (Fig. 15.7b). Increasing the incubation period to 12 h did not have marked improvement in penetration depths. CLSM images (Fig. 15.7e) show higher intensities and slightly higher penetration up to viable epidermis achieved for both the dyes. Although distribution of Alexa Fluor-488® appears uniform for both 6 and 12 h diffusion samples, distribution of lipophilic dye DiI was not uniform for 12 h samples. For skin treated with liposomal formulations containing 1 % terpenes, much higher intensities were observed for both the fluorescent dyes in the SC (Fig. 15.7c, f). For sample with 6 h incubation, very weak fluorescence for Alexa Fluor-488® while weak fluorescence for DiI was observed in the viable epidermis. For 12 h incubation samples, fluorescence intensity scores were bright-fluorescence at SC for both the dyes and moderate fluorescence intensities were observed in the viable epidermis. Surprisingly, the reddish fluorescence continued from the epidermis toward the dermis, indicating a diffusion of the lipophilic marker. It was observed that liposomal formulations containing terpenes as penetration enhancers were able to deliver relatively higher amounts of fluorescent labels into the SC, epidermis, and to a small extent the dermis. In this study, we clearly demonstrated the ability of CLSM to simultaneously image two fluorescent dyes.


Fig. 15.7
Cross-sections of human abdominal skin treated with formulations containing two fluorescent markers, DiI and Alexa Fluor 488®. The formulations were applied without occlusion for 6 h (a–c) and 12 h (d–f). (a, c) Hydro-alcoholic solution of DiI and Alexa Fluor 488, (b, e): double-labeled liposomes without terpenes (c, f): double-labeled vesicles containing 1 % terpenes. The bar represents 10 μm
15.2.5 Tracking the Penetration of Fluorescence Labels into Hair Follicles
15.2.5.1 Tracking of Fluorescently Labeled Cyclosporin A into Rat Hair Follicles
CLSM has been successfully used to track the follicular penetration of drugs. Topical application of the CF-loaded liposomes has been reported to significantly increase the accumulation of CF in the pilosebaceous units compared to other non-liposomal formulations (Lieb et al. 1992). Using CLSM, Patzelt et al. demonstrated that follicular penetration for nanoparticles was size dependent (Patzelt et al. 2011).
The effect of terpenes as penetration enhancers in liposomes for targeting hair follicles was investigated using Fl-CyA vesicles (as mentioned above), with and without terpenes, in rat skin. Figure 15.6 also depicts the role of the pilosebaceous unit in the penetration of drugs into the skin. A bright fluorescence was observed in the pilosebaceous units (bright green fluorescent regions in dermis in Fig. 15.6b, c identified as the hair shaft, a part of the pilosebaceous unit) for both formulations, with and without PE. The fluorescence was also visualized in the outer root sheath of the hair shaft (Figs. 15.6b–c and 15.8) (Verma 2002). The images presented here demonstrate that the liposomal vesicles can enter the pilosebaceous unit and deliver their content to the hair follicle and possibly to the hair bulb. The importance of this was stressed upon by the fact that ethanolic solution of Fl-CyA failed to deliver any fluorescence into the skin. These CLSM results were supported by in vivo studies with Dundee Experimental Bald Rats model (Verma et al. 2004) and other published reports (Agarwal et al. 2000; Bohm and Luger 1998; Lieb et al. 1992; Niemiec et al. 1995). The CLSM investigations enabled us to visualize the accumulation of fluorescent label in the pilosebaceous units, which is not possible with tape stripping.


Fig. 15.8
Fl-CyA liposomes treated rat skin cross-section demonstrating the role of pilosebaceous units in the penetration of substance into the skin. The bar represents 10 μm. (a) Fluorescence micrograph and (b) transmission-mode image of the cross-section
15.2.5.2 Accumulation of CF and N-Rho-PE Loaded Liposomes in the Human Hair Follicles
In this study, liposomal formulations containing CF and N-Rh-PE were prepared (Verma 2002). Liposomal formulations were able to deliver both the hydrophilic and lipophilic fluorescent labels to the human hair follicles as seen in Fig. 15.9a, b. Studies represented in Figs. 15.6, 15.8, and 15.9 demonstrated that the presence of hair follicles plays a significant role in the skin penetration of drugs, as well as that the presence of penetration enhancers helps the formulation in enhancing the follicle delivery of drugs.


Fig. 15.9
Fluorescence micrographs showing (a) CF delivery to hair follicles and (b) high-resolution micrograph showing rhodamine delivery to hair follicle following topical application of fluorescent liposomes
15.2.6 The Efficacy of Dermaroller® to Enhance Penetration into the Skin
CLSM was also used to investigate the efficacy of the micro-perforation devices, Dermaroller® (Horst Liebl ETS, France), in increasing the skin penetration of a fluorescent lipophilic model compound DiI encapsulated in liposomes (Verma 2002). Three types of Dermarollers® were tested in this study, namely, Dermaroller® C8 0.13–15°, M8 1.5–15°, and M8 1.5–30°. Control was provided by the skin not treated with Dermarollers®.
Bright intensity of fluorescence was observed in SC for all samples treated with Dermaroller and for control (Fig. 15.10). For control samples, however, fluorescence intensities in the viable epidermis and dermis were very low. For Dermaroller® C8 0.13–15°, highest fluorescence was observed in the SC and fluorescence intensities rapidly decreased in the viable epidermis and dermis (Fig. 15.10b). Dermaroller® C8 0.13–15° was intended for delivering drugs to the SC whereas Dermaroller® M8 1.5–15° and M8 1.5–30° were optimized for delivering drugs to the deeper regions of skin. Both M8 Dermarollers® did show very high fluorescence intensities in the dermis region (Fig. 15.10c, d). We observed that samples treated with both M8 Dermarollers® showed only a weak fluorescence in the viable epidermis region and showed weak lateral diffusion of the fluorescent label from the pores. This might be because Dermaroller® was able to deliver the liposomes into the deeper layers quickly during application of the Dermaroller but after the application, liposomes probably were not able to diffuse to a great extent from the surface of the skin to the deeper layers in the skin. In this study, we emphasized on the spatial distribution of the fluorescent label which helped us to get a better understanding of the mode of action of the micro-perforation device, Dermaroller®.


Fig. 15.10
Fluorescent micrographs of cross-sections of human abdominal skin pretreated with Dermaroller® and incubated on a Franz diffusion cell with liposomes containing lipophilic fluorescent label DiI. The liposomes were applied without occlusion for 3 h. (a) Control; (b) Model C 8 0.13–15°; (c) Model M 8 1.5–15°; (d) Model M 8 1.5–30°
15.3 Two-Photon Fluorescence Microscopy
15.3.1 Principle of Two-Photon Fluorescence Microscopy
As seen from aforementioned studies, CLSM is a very good technique for the imaging of biological samples; however, it suffers from some disadvantages. One of the most important limitations of the CLSM is that because only a small fraction of the incident light reaches the detector, relatively high intensities of light are required to achieve high signal to noise ratios. This results in high total energy transfer to the specimen during imaging. Also, the laser beam excites the fluorophores in the specimen above and below the focal plane that is under investigation and this can cause severe photo bleaching, photo damage, and even dehydration of the biological specimen. Many fluorophores generate free radicles or singlet oxygen when excited which can be toxic if imaging living cells or tissue. CLSM also suffers from low axial resolution especially when imaging deep within a thick tissue. The poor axial resolution results from the scattering of light by the specimen itself, which can lead to detection of light that is not generated from the observed focal plane. Also CLSM employs short wavelength excitation sources and shorter wavelengths are scattered stronger (scattering intensity is inversely proportional to fourth power of the wavelength) hence these excitation sources are not very efficient at deep tissue imaging.
Due to limitations in deep tissue imaging, when adopting a CLSM for imaging skin, the sample must be cryo-sectioned and this is not always possible and desirable.
Multiphoton excitation phenomenon was proposed in 1931; however, Kaiser and Garret were able to validate it only in 1961 after the development of lasers which were sufficiently powerful to generate the high photon flux required for two-photon excitation. Figure 15.11a explains the energy transfer during one-photon and two-photon excitation. In single-photon fluorescence, the fluorescence photon is generated when a high energy photon is incident on the fluorophore, which results in raising the energy level of one of the electrons to an excited state. In two-photon excitation, if two low energy photons arrive together within 10−18 s of one another, the combined energy transfer of the two low energy photons is sufficient to raise the same electron to a higher energy level (Dunn and Young 2006). A low energy photon is too weak to illicit the two-photon fluorescence phenomenon in the fluorophore. Hence, in order to increase the probability of simultaneous incidence of photons a laser with very high photon flux is required. Setup of a two-photon microscope is quite similar to that of a confocal scanning microscope with two major differences. One is the light source, two-photon microscopes use a tunable Ti-sapphire high frequency pulsed laser which has a pulse length of approximately a few hundred femtoseconds. The second important difference is the lack of any pinholes in front of the detector to avoid detecting scattered light from out of focus plane.


Fig. 15.11
(a) Jablonski diagram elucidating the differences between single photon fluorescence and two photon fluorescence phenomenon. (b) Excitation volume in one-photon and two-photon fluorescence phenomena
One of the important advantages of 2-PFM is that total energy delivered to the specimen is much lower as compared to a CLSM. Also the two-photon excitation phenomenon occurs only at a very small focal volume (Fig. 15.11b) and as a result fluorophores in a very small volume of the specimen are excited, which reduces the probability of photo-bleaching, photo-damage. In addition, no confocal pinhole aperture is required to block the scattered light from out of focus planes. The near-infrared (NIR) light source used in a 2-PFM is very efficient at imaging deeper regions of the biological samples, as it is least scattered and absorbed by the biological samples. These advantages mean that the skin samples can be studied without cryofixing and cutting.
Disadvantages of a two-photon microscope include a relatively high price of the ultra-short pulsed Ti-sapphire lasers and the fact that they require an elaborate cooling system. Also, 2-PFM has lower lateral resolution compared to CLSM, but in practice the difference in their resolution is not significant.
15.3.2 Application of Two-Photon Microscopy in Skin Penetration Experiments
Carrer et al. used 2-PFM to study the architecture and physical properties of the pig skin epidermis and permeability of different liposomal formulations in the pig skin (Carrer et al. 2008). In this study, authors were able to easily distinguish different layers of the pig epidermis based on their morphological differences without any significant sample preparation. To study the hydration/polarity of different layers of the epidermis they used Laurdan generalized polarization (Laurdan-GP) values as an indicator of polarity. As expected, the Laurdan-GP values were highest for SC and decreased for deeper layers of the skin indicating an increase in fluidity or hydration. The authors report an interesting observation in the intercluster region (canyons or wrinkles as in human skin), the Laurdan-GP values in the canyons were quiet high, comparable to that of SC, and they did not change with depth (from surface of SC to dermis), indicating that canyons might show environment similar to SC. To study the penetration of liposomal formulations, Lissamine rhodamine B 1,2-dihexadecanoyl-sn-glycero-3-phosphoethanolamine was incorporated in the liposomal membrane. After 16-h diffusion, image stacks were collected and penetration was measured in the form of ratio of fluorescence intensity of rhodamine and autofluorescence of the skin. Authors report that the liposomes with lower lipid content (10 mg/ml) were able to deliver more fluorescent label to the lower parts of viable epidermis compared to the formulations with higher lipid content (25 and 50 mg/ml).
van den Bergh et al. elucidated the mechanism of interactions of elastic and rigid liposomal vesicles with human skin (van den Bergh et al. 1999). Using 2-PFM and different electron microscopic techniques they studied interactions between skin and various elastic liposomal formulations to understand the modulation of the skin barrier. They observed that fluorescently labeled elastic vesicles primarily affected the intercellular lipid lamellae of the SC, while the underlying layers of the viable epidermis remained relatively unchanged. This effect was visualized using 2-PFM, in the form of small penetration pathways that were confined to the SC only. Authors also reported that the penetration enhancing ability of elastic vesicles was dependent on whether vesicles were applied with or without occlusion.
Oleic acid is a good penetration enhancer and has been found to increase transdermal penetration of both hydrophilic and hydrophobic drugs by modulating the intercellular lipid domains in the SC. Yu et al. used a high-speed two-photon microscope with dual channels capable of simultaneous monitoring of autofluorescence of skin and fluorescence from rhodamine as a model drug (Yu et al. 2003). Treatment of human skin with oleic acid as a penetration enhancer was found to induce increased intra-corneocyte diffusion of the hydrophilic model drug (sulforhodamine-B), while causing localization of the hydrophobic model drug (Rhodamine-B hexyl ester) to the intercellular region. Hence, Yu et al. provided evidence that for chemical penetration enhancers, intra-corneocyte diffusion is an important enhancement mechanism along with fluidization of intercellular lipid domains, and physicochemical properties of the drug would determine which pathway predominates in the penetration enhancement.
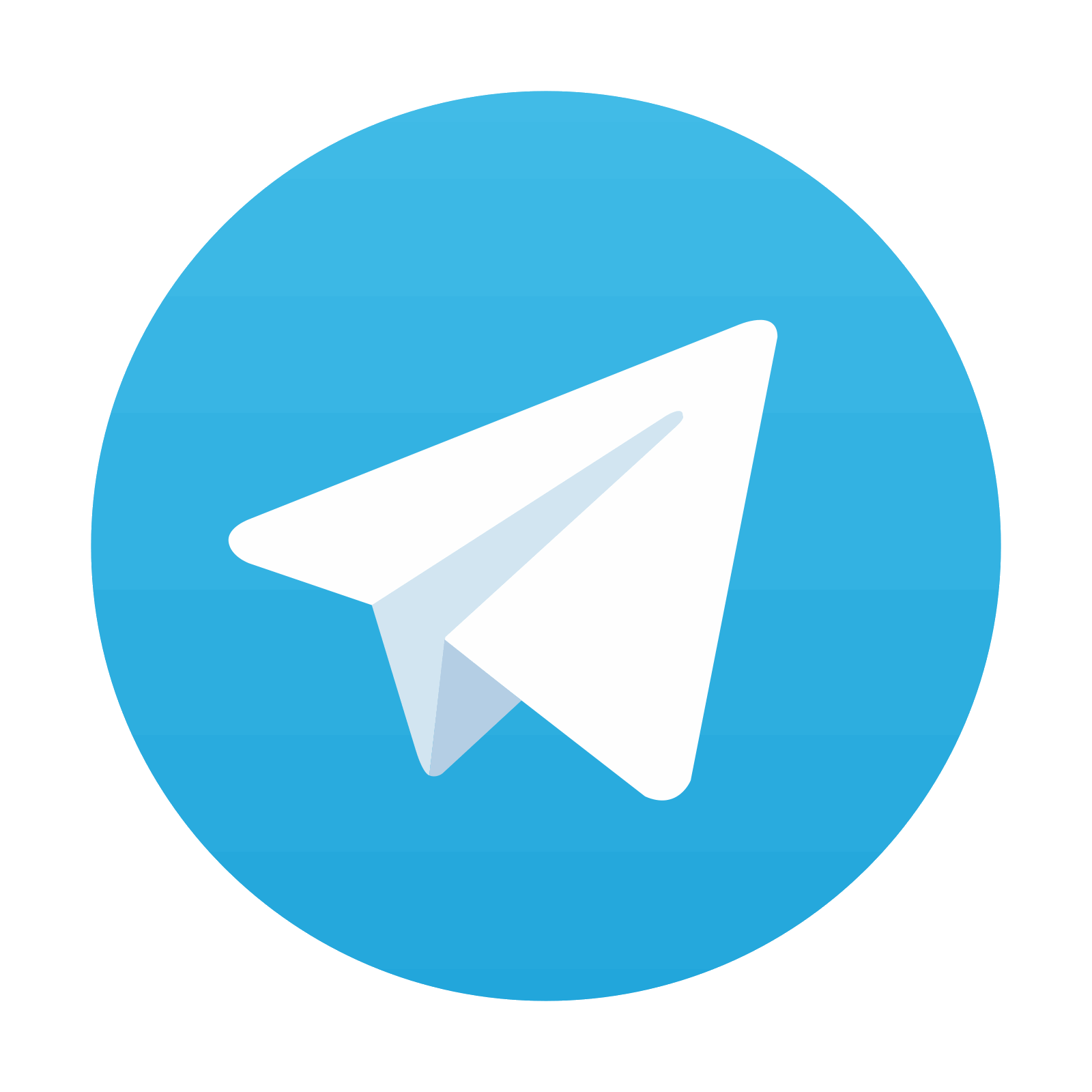
Stay updated, free articles. Join our Telegram channel
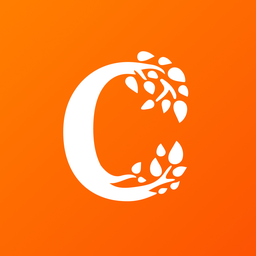
Full access? Get Clinical Tree
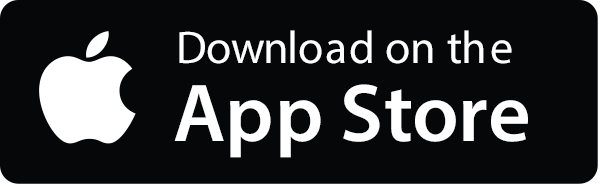
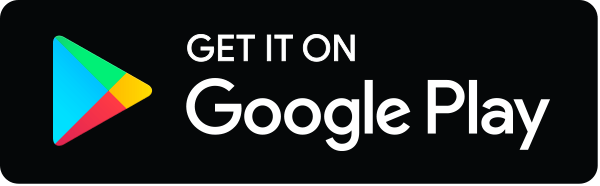