Fig. 16.1
Volunteer being imaged with a hand-held in vivo reflectance confocal microscope (Vivascope 3000®, Lucid Inc., USA)
16.2.1 Characterization of Percutaneous Drug Delivery
Percutaneous drug delivery encompasses many approaches from the simple passive diffusion of small compounds through to the active delivery of much larger macromolecules. The need for enhanced percutaneous penetration has been well-established, with a range of chemical and physical techniques being developed. CLSM has played and is continuing to play an important role in validating these techniques – visualizing the delivery and distribution within the skin (Prow et al. 2010; Fernando et al. 2010). For example, in Fig. 16.2 we show the difference in delivery profiles between topical and microneedle enhanced delivery of sodium fluorescein within volunteers. By averaging the signal intensity per optical section we were able to ‘map’ the delivery profiles in a cross-sectional manner. This is a powerful technique because it clearly shows the influence of enhanced percutaneous delivery giving insight into distribution and penetration of the payload.


Fig. 16.2
Images showing the in vivo human delivery of sodium fluorescein (NaF) with and without microneedles (MN) using dermoscopy (top panels) and confocal laser scanning microscopy (middle panels). The bottom panels are a cross-sectional heat map representation of the average delivery profile (represented by rectangular box). Arrow indicates a microneedle deposition site
Lademann et al. (2003) investigated the use of a hand-held CLSM designed for dermatology applications (Optiscan Stratum™, Optiscan Ltd., Australia) (Lademann et al. 2003). The device was designed to be relatively simple and portable for ease-of-use within a clinical setting. The excitation source consisted of an argon ion laser with a wavelength of 488 nm specific for in vivo dyes curcumin or fluorescein. The two dyes were applied to male and female volunteers in either ethanol or sunscreen emulsion. Fluorescein application resulted in visualization of the different skin layers – stratum corneum, stratum granulosum, stratum basale and although of low resolution, the papillary dermis (Fig. 16.3). Lademann et al. hypothesized that using their CLSM system in conjunction with the fluorescein/ethanol solution, different skin layers could be distinguished between a healthy or diseased state (Lademann et al. 2003).


Fig. 16.3
Confocal laser scanning microscopy of sodium fluorescein within the different layers of volunteer skin: (a) stratum corneum, (b) stratum granulosum, (c) stratum basale, and (d) papillary dermis (Adapted from Lademann et al. (2003))
The curcumin/sunscreen emulsion was used to investigate potential penetration pathways and distribution of sunscreens within the skin. It was determined that the sunscreens accumulated around the edges of the corneocytes. Initially only the skin surface could be detected, however over time (20 min) three cell layers of the stratum corneum became visible. Additionally, the curcumin solution accumulated around the hair follicles and within the sweat glands. Interestingly, Lademann et al. were able to distinguish between active and passive sweat glands based on the distribution of the dye in or around the gland (Lademann et al. 2003). This study demonstrated that hand-held CLSMs have the potential for non-invasive clinical imaging.
The Optiscan Stratum™ has also been used as a validation tool assessing the penetration of microneedles within volunteers (Mukerjee et al. 2004; Bal et al. 2010a, b). Mukerjee et al. developed hollow and solid microneedles up to 200 μm in length with various diameters (Mukerjee et al. 2004). Solid microneedles were applied to the skin, near the first knuckle of the thumb followed by application of a 0.1 % sodium fluorescein solution. The application of the sodium fluorescein solution without microneedles resulted in no dye solution penetrating through the stratum corneum. CLSM assessment of microneedle application resulted in the visualization of successful microneedle penetration to a depth of 160 μm. Although no investigation was done on dye distribution and diffusion within the skin, the study showed that the application of Optiscan Stratum™ was a suitable technique for investigating the microneedle enhanced delivery of fluorescein.
In 2010, Bal et al. conducted a more in-depth microneedle study in volunteers using the delivery of fluorescein as a visual guide for characterizing the microneedle conduits and their closure over time (Bal et al. 2010a). Bal et al. also used the Optiscan Stratum™. The microneedle array consisted of 5 microneedles, 300 μm in length, 120 μm in diameter and a spacing of 2 mm. Bal et al. investigated the two techniques of fluorescein – microneedle application by either applying the dye to the skin prior to or after microneedle administration. After each application CLSM was used to characterize the microneedle conduit. Dye application post microneedle piercing resulted in significantly higher signal than dye application prior to microneedles. Bal et al. concluded that the conduits closed within 10–15 min after microneedle application and that a depth of 150 μm was achieved (suitable for macromolecules to reach the viable epidermis (Fig. 16.4a, b)).


Fig. 16.4
Microneedle-enhanced delivery of sodium fluorescein in volunteers. (a) Confocal laser scanning microscopy of microneedle delivery 5, 10 and 15 min post application at two depths (surface and 20 μm below the surface). (b) Comparison maximum depth of dye diffusion over time between sodium fluorescein application before or after microneedle insertion (Adapted from Bal et al. (2010a))
More recently, Vergou et al. compared the use of transepidermal water loss (TEWL) and CLSM for the in vivo characterization of the stratum corneum barrier in volunteers (Vergou et al. 2012). Once again, this study was done using the Optiscan Stratum™. TEWL is a relatively standard technique used to characterize the integrity of the epidermal barrier following disruption with percutaneous penetration enhancers. However, TEWL measurements can be influenced by internal and external factors such as humidity, temperature and air convection. Additionally, TEWL is not reliable post drug application where the drug or chemical enhancer solution is still on the skin’s surface. Vergou et al. assessed the barrier integrity prior to and post treatment of dry skin (without disease) with gel and oil products (Valeo Holundergel and Valeo Dehnungspflegeoel, L’estétic GmbH, Germany) designed to rehydrate the skin following radiotherapy treatment (Vergou et al. 2012). The gel was designed to penetrate the skin – increasing hydration – followed by the oil which formed a protective layer on the skin’s surface to maintain hydration. The products were applied twice daily on the volar forearm over a 4-week period. No treatment was done to the other arm.
Assessment was done prior, 2 weeks after and 4 weeks after application. The initial TEWL values were standardized to 100 % between volunteers and it was determined that for all volunteers the TEWL for untreated skin remained constant (99 ± 17 %). The TEWL reading for the treated arm increased by 21 ± 27 % suggesting disruption of the epidermal barrier. However, stratum corneum hydration and elasticity experiments resulted in an average increase of 20 % and 10 %, respectively, indicating improved integrity of the epidermal barrier. CLSM showed a similar trend to the hydration and elasticity studies, where a distinct improvement in the integrity of the stratum corneum was observed. Prior to treatment the corneocytes showed an ‘irregular mountainous structure’. After the 4-week treatment, the corneocytes formed a flat honey-comb pattern surrounded by intact lipid layers. Vergou et al. hypothesized that even though TEWL is non-invasive, when placed on the skin surface it disrupted the protective oil layer resulting from the treatment. Accumulated water from the gel treatment was then detected by TEWL (Vergou et al. 2012). This resulted in higher TEWL readings, which inferred a greater disruption of the stratum corneum, when in reality visual microscopic inspection showed that the stratum corneum was intact.
Overall Vergou et al. and others have shown the utility and importance of visual methods when characterizing topically applied formulations in vivo. The application and instrumentation of the described in vivo fluorescence microscopes are relatively simple to use and result in information relating to drug distribution within skin and insight into their mechanism of action. A benefit of using the Optiscan Stratum™ in relation to the investigation of percutaneous enhanced penetration is that tissue and cellular disruption can be visualized. Understanding the change in skin integrity is important for characterizing the chemical and/or physical methods used to deliver drugs. However, the limitation of the current technique is that a fluorescent solution is required to visualize the skin layers (in the previous cases, sodium fluorescein). Depending on the depth required, the fluorescent solution is injected into the skin or topically applied, potentially influencing the drug of interest.
16.2.2 Reflectance Microscopy for the Assessment of Therapeutic Changes in the Skin
Reflectance confocal microscopy (RCM) is an alternate approach to fluorescence-based CLSMs. RCM can especially be useful to characterize skin integrity and disruption as well as the change in disease state of the skin following therapeutic treatment (Ardigo et al. 2010; Segura et al. 2011; Ulrich et al. 2009). Various studies have been published characterizing the morphological changes seen in the skin over time, including studies looking at wound healing.
RCM utilizes a near-infrared diode laser as a monochromatic and coherent light source. The light source is reflected (backscattered) off the sample and passes through a pinhole aperture to the detector. RCM results in quasi-histological resolution because backscattering occurs at the edges of two biological materials with different refraction indexes – thus distinguishing cellular structures. RCM can be used for assessing structural changes within the skin in addition to serial imaging of the same site over time (Wurm et al. 2012). Furthermore, the use of near-infrared laser sources results in relatively deep imaging down to the dermo-epidermal junction. The high cellular and structural resolution images obtained from RCM make it a valuable tool when assessing percutaneous penetration enhancers. RCM has the potential of providing information on the change in skin integrity post chemical and/or physical enhancement and unlike the previous fluorescence-based technique described it does not require a dye solution.
RCM is also used as a qualitative and quantitative technique where differences can be observed relating to the cellular and extracellular structures within skin. For example, we have investigated in the Prow group, the differences in skin from two age groups, 20–30 years and 50–60 years, respectively, using RCM (Fig. 16.5). The RCM images in Fig. 16.5b–d, f–h correspond to three distinct skin layers of interest (stratum corneum, stratum spinosum and dermo-epidermal junction). We have reported in detail and scored the RCM features associated with photo-ageing on the forearm of the two age groups (Wurm et al. 2012). We concluded that 15 statistically significant RCM features, such as furrow width/shape, keratinocyte irregularity/disarray and dermal papillae morphology could be used to quantify photo-ageing in forearm skin (Wurm et al. 2012).


Fig. 16.5
Clinical photographs and their corresponding reflectance confocal microscopy (RCM) images. (a) and (e) show representative clinical photographs of volunteers from the two age groups, 20–30 years and 50–60 years, respectively. (b–d) and (f–h) show the corresponding RCM images of volunteers (a) and (e), respectively. (b) and (f) – stratum corneum, (c) and (g) – stratum spinosum, (d) and (h) – dermo-epidermal junction (Prow, Raphael and AR Soyer, unpublished 2012)
One particular area that has seen much attention using RCM is the characterization and pre- and post-topical treatment of pre-cancerous and cancerous skin lesions (actinic keratosis [AK], basale cell carcinoma [BCC], squamous cell carcinoma [SCC] and melanoma). Segura et al. investigated the efficacy of photodynamic therapy (PDT) using methyl-aminolevulinate (MAL) on the treatment of BCCs (Segura et al. 2011). Six patients were treated with 1–3 cycles of MAL-PDT with each cycle consisting of two sessions of MAL-PDT 7 days apart. To enhance MAL penetration into the skin, debridement of the BCC surface was done with a curette followed by a 3 h application of MAL. The patients were assessed 1 week and 3 months post treatment using RCM with consecutive follow-ups (without RCM) every 6 months for 3 years. The RCM used was a commercially available instrument designed specifically for in vivo applications (Vivascope 1500®, Lucid Inc., USA). The system consists of a 830 nm diode laser and a 30× water immersion lens facilitating a horizontal optical resolution of 2 μm and vertical resolution of 5 μm. It was determined that RCM analysis was suitable for characterizing whether lesions underwent partial or complete remission. In these cases not all treated lesions underwent complete remission. However, those that did were characterized by the presence of normal dermal papilla and collagen fibres (Fig. 16.6).


Fig. 16.6
Clinical, dermoscopy and RCM images of a 4 mm pigmented basal cell carcinoma on the abdomen of a 25-year-old woman. (a) Clinical and dermoscopy (inset) images of the lesion before photodynamic therapy. (b) A 4 × 4 mm reflectance confocal microscopy image (RCM) of the lesion prior to treatment. (c) Magnified image (0.5 × 0.5 mm) of the lesion at the dermo-epidermal junction. (d) Clinical and dermoscopy (inset) images of the lesion after two cycles of photodynamic therapy. (e) A 4 × 4 mm RCM image of the lesion after treatment showing reduction in tumour size. (f) Magnified image (0.5 × 0.5 mm) of the lesion after treatment at the dermo-epidermal junction showing the presence of normal dermal papilla (Adapted from Segura et al. (2011))
Torres et al. published a similar study treating basale cell carcinomas (BCCs) with 5 % imiquimod (Torres et al. 2004). Patients applied imiquimod 5 times daily for 2, 4 or 6 weeks, with RCM analysis at the 6-week follow-up. Torres et al. also used a Lucid Inc., USA, based RCM system designed for in vivo investigations. Whereas Segura et al. characterized the BCCs based on the skin morphology within the dermo-epidermal junction and dermis, Torres et al. focused on the keratinocyte structure and cytoplasm-to-nucleus ratio within the epidermis (Torres et al. 2004). BCCs were characterized as having elongated keratinocytes with a high cytoplasm-to-nucleus ratio compared to normal skin, which consisted of a lower ratio and regular honey-comb pattern of the cells (Fig. 16.7). Even though in this study, RCM was seen as a useful technique evaluating tumour regression in vivo without the need of surgery, there were still some limitations. The investigators felt that the technique was time-consuming (although far quicker than an invasive surgery approach). There were also technical difficulties due to movement of the relatively large scanning head, skin contact angle and maintaining contact with the skin during imaging. These limitations can be overcome with practice, however it does prevent implantation of such in vivo systems especially in laboratories that are familiar primarily with in vitro and ex vivo investigations. However, it should be noted that the RCM used was a much earlier model available from Lucid Inc. (Vivascope 1000®) and since then Lucid Inc. have developed small hand-held systems with greater ease-of-use and flexibility (Fig. 16.1).


Fig. 16.7
Reflectance confocal microscopy (RCM) images of a basal cell carcinoma (BCC) before and after treatment with imiquimod. (a) BCC morphology prior to treatment showing elongated cells with a high cytoplasm-to-nucleus ratio (nucleus low signal, cytoplasm high signal). (b) BCC morphology 2 weeks after treatment showing regular honey-comb pattern and normal cytoplasm-to-nucleus ratio (Adapted from Torres et al. (2004))
In summary, CLSM systems are increasing in their use as non-invasive techniques for percutaneous drug delivery. Although fluorescence-based systems are limited mainly to in vitro and ex vivo applications, RCM is being utilized clinically much more due to its ability to resolve cellular and structural morphology within the skin pre and post drug application. With an increase in biocompatible fluorophores, user-friendly equipment and cost-effective instruments, it is expected that in vivo CLSM systems will continue to be incorporated into pharmaceutical and percutaneous research.
16.3 Applications of Multiphoton Microscopy
Multiphoton microscopy (MPM) is a deep tissue imaging technique that is predominantly used for non-linear two-photon excitation of fluorophores. MPM can additionally be used for three-photon excitation as well as second and third harmonic generation of biological structures. These features, combined with the fact that MPM can result in single photon sensitivity with submicron resolution has led it to being an important microscopic technique with the highest resolution in the area of non-invasive clinical imaging (Fig. 16.8) (Konig et al. 2006; JenLab GmbH).


Fig. 16.8
In vivo multiphoton microscopy system (MPTflex™, JenLab GmbH, Germany) (Reprinted from JenLab website (JenLab GmbH))
Two-photon excitation works on the theoretical principal developed by Maria Göppert-Mayer, where two photons of similar energy interact with a molecule (fluorophore) resulting in excitation equivalent to the absorption of a single photon with twice the energy (Fig. 16.9) (Zipfel et al. 2003; Prow 2012). The probability of successful two-photon interaction (absorption) can be measured quantitatively by the two-photon cross-section (σ 2p). The two-photon cross-section is referred to as Göppert-Mayer (GM) where 1 GM is equivalent to 10−50 cm4s. Instead of measuring σ 2p, which is quite complex, the product of the fluorescence quantum yield (φ F) and absolute two-photon cross-section is calculated, which is the two-photon ‘action’ cross-section (φ F σ 2p) (Zipfel et al. 2003). To determine the optimal wavelength for two-photon excitation, it is useful to have an understanding of the change in φ F σ 2p values with wavelength (two-photon excitation spectra). This is because a molecule’s maximum single photon excitation does not always correspond to two photon excitation with similar energy (in particular symmetrical and intrinsic molecules). Therefore, when selecting fluorescent tracers for analysis of percutaneous penetration with multiphoton microscopy it is important to have an understanding of φ F σ 2p.


Fig. 16.9
Simplified schematic of the optical layout for multiphoton microscopy and energy transfer diagrams. Tuneable 80 MHz titanium sapphire laser sources are most commonly used for MPM. The light source is then raster scanned over the imaging area using scanning galvanometers. The dichroic mirror and objective lens then pass the excitation beam to the sample. The emission is collected and passed to one or more detectors. Photomultiplier tubes and time-correlated single-photon counting detectors can be used depending on application. Single, two-photon and second-harmonic generation Jablonksi diagrams are showed on the right (Reprinted from Prow (2012))
For successful excitation to occur, the photons need to interact with the molecule almost simultaneously (approx. 10−16 s). Therefore, the probability of successful two-photon excitation is greatest within the beam area within the focal plane. Outside of the focal plane the probability drops significantly with negligible fluorescence emission. To further increase the probability of two-photon excitation and generate sufficient fluorescence for imaging, high laser powers are required. However, high laser powers are detrimental to biological samples. To address this problem, MPMs utilize a pulsed laser (most commonly being a mode-locked titanium sapphire laser), which produces approx. 80 million pulses per second with a pulse duration of approx. 100 fs. The pulsed laser results in a significant increase in the probability of two photon excitation while keeping the average power relatively low. These laser power peaks contain enough dense photons to create fluorophore excitation and also retain the average power fairly low.
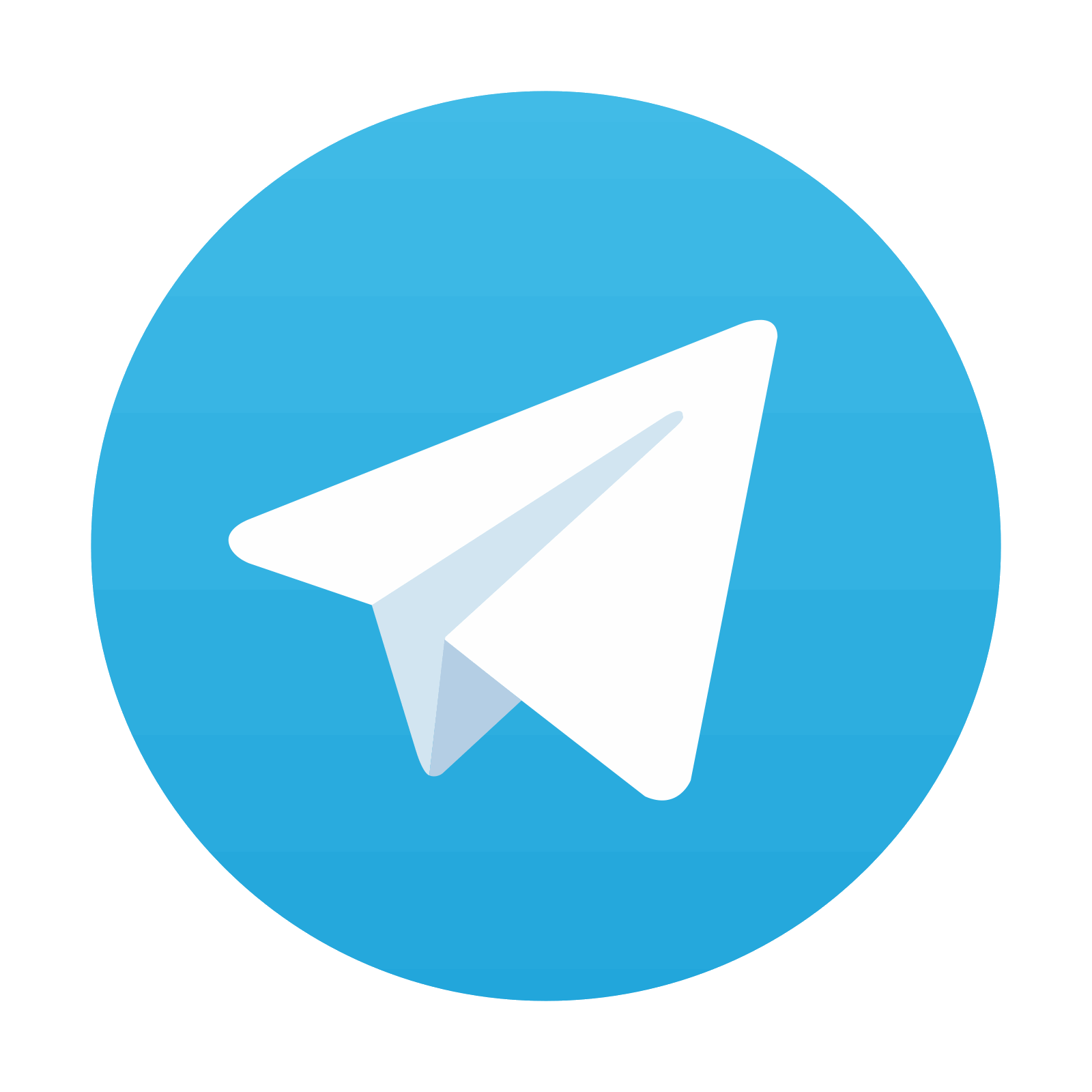
Stay updated, free articles. Join our Telegram channel
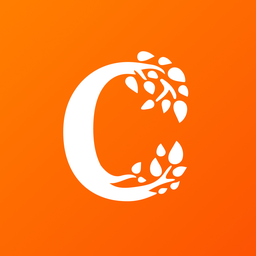
Full access? Get Clinical Tree
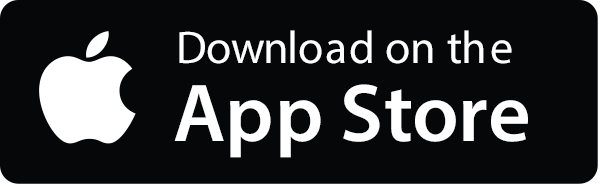
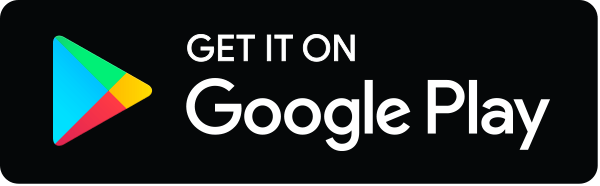