Biology of Eccrine and Apocrine Glands: Introduction
|
Development of Eccrine Sweat Glands
Humans have approximately 2–4 million sweat glands.1 Sweat glands are found over nearly the entire body surface, and are especially dense on the palms, soles, forehead, and upper limbs.2 Analgen of eccrine sweat glands first appear in the 3.5-month-old fetus on the palms and soles (see Chapter 7), then develop in the axillary skin in the fifth fetal month, and finally develop over the entire body by the sixth fetal month.3 The analog of the eccrine sweat gland, which developed from the epidermal ridge, is double layered, and develops a lumen between the layers between the fourth and eighth fetal months. By the eighth fetal month eccrine secretory cells resemble those of the adult; by the ninth fetal month myoepithelial cells form.
Anatomy and Function of Eccrine Sweat Glands
Secretory Coil
The secretory coil contains three distinct cell types: (1) clear (secretory), (2) dark (mucoid), and (3) myoepithelial.4 The clear and dark cells occur in approximately equal numbers but differ in their distribution (Fig. 83-1). While the dark cells border the apical (luminal) surfaces, the clear cells rest either directly on the basement membrane or on the on the myoepithelial cells. The clear cells directly access the lumen by forming intercellular canaliculi (Fig. 83-2). Spindle shaped contractile myoepithelial cells lie on the basement membrane and abut the clear cells. The adult secretory coil is approximately 2–5-mm long, and approximately 30–50 μm in diameter. Heat accumulation results in larger sweat glands and ducts, and their dimensions, in turn, correlate with enhanced sweat output.5 Clear cells contain abundant mitochondria and an autofluorescent body, called the lipofuscin granule, in the cytoplasm. The clear cell plasma membrane forms many villi. The clear cell secretes water and electrolytes. Dark cells have a smooth cell surface and contain abundant dark cell granules.4 The function of dark cells are unknown. Myoepithelial cells contain actin filaments6 and are contractile,7,8 producing pulsatile sweat.
Figure 83-1
Light photomicrograph of the secretory coil of an acetylcholine-stimulated monkey palm eccrine sweat gland. A 1-m thick section was cut from an Epon-embedded specimen and stained with methylene blue. Inset: A higher-power view of the area marked by the square. CC = clear cell; DC = dark cell; ICC = intercellular canaliculi; Lu = lumen; MC = myoepithelial cell.
Figure 83-2
Electron micrograph of the secretory coil of a human eccrine sweat gland. B with arrow = basal lamina; other symbols are the same as in Fig. 83-1.
Duct
The eccrine sweat duct consists of an outer ring of peripheral or basal cells and an inner ring of luminal or cuticular cells. It seems that the proximal (coiled) duct is functionally more active than the distal straight portion in pumping Na+ for ductal Na+ reabsorption, because Na+, K+–adenosine triphosphatase (ATPase) activity and the number of mitochondria are higher in the proximal portion (Fig. 83-3).4,7,9,10 In contrast, the luminal ductal cells have fewer mitochondria, much less Na+, K+-ATPase activity, and a dense layer of tonofilaments near the luminal membrane, which is often referred to as the cuticular border. The cuticular border provides structural resilience to the ductal lumen, which may dilate whenever ductal flow of sweat is blocked. The entire structural organization of the duct is well designed for the most efficient Na+ absorptive function. The luminal membrane serves as the absorptive surface by accommodating both Na+ and Cl− channels, and the basal ductal cells serve in Na+ pumping by providing maximally expanded Na+ pump sites and efficient energy metabolism. The lumen and the duct contain β-defensin, an antimicrobial, cysteine-rich, low-molecular-weight peptide.11,12 In the epidermis, the duct spirals tightly upon itself.
Figure 83-3
Drawing of the ultrastructure of the eccrine duct and secretory coil and the localization of Na+, K+–adenosine triphosphatase (ATPase). The thick lines indicate the localization of Na+, K+-ATPase. BM = basement membrane; C = clear cell; D = dark cells; IC = intercellular canaliculi; M = myoepithelial cell; Mc = mitochondria.
The preoptic hypothalamic area plays an essential role in regulating body temperature: local heating of the preoptic hypothalamic tissue activates generalized sweating, vasodilatation, and rapid breathing, whereas local cooling of the preoptic area causes generalized vasoconstriction and shivering. The elevation of hypothalamic temperature associated with an increase in body temperature provides the strongest stimulus for thermoregulatory sweating responses, while cutaneous temperature exerts a weaker influence on the rate of sweating.13 On a degree-to-degree basis, an increase in internal temperature is about nine times more efficient than an increase in mean skin temperature in stimulating the sweat center. The local temperature effect is speculated to be due to increased release of periglandular neurotransmitters.
The sweating in menopausal “hot flashes” reinforces the concept of a central hypothalamic mechanism for thermal sweating, but also shows that the response of individuals to the same changes in core temperature can vary. Although hormonal factors influence sweating during menopause, excessive sweating does not correlate simply with hormonal levels. Instead, menopausal hot flashes seem to be due to a hypersensitive brain response (particularly the hypothalamus, but perhaps the insula, anterior cingulate, amygdala, and primary somatosensory cortex as well). In asymptomatic menopausal women and premenopausal women, the core temperature can change up to 0.4°C (33°F) without eliciting a response. In symptomatic postmenopausal women, changes as small as 0.1°C (32°F) trigger peripheral vasodilation and sweating. Why the brain is hypersensitive to small changes in core temperature is poorly understood, but increased levels of brain norepinephrine appear to influence the response to small changes in core temperature through their action on α2-adrenergic receptors in the brain; higher levels of the norepinephrine metabolite 3-methoxy-4-hydroxyphenylglycol have also been found in symptomatic menopausal women than in asymptomatic women. Decreased norepinephrine release is postulated as the mechanism by which clonidine relieves hot flashes in symptomatic women. Decreased core temperature may be the reason that women with decreased body mass index tend to have fewer symptoms, even though their estrogen levels probably are lower than those in women with increased body mass index. Levels of estrogen, luteinizing hormone, and β-endorphins also were originally thought to influence hot flashes, but later studies have suggested no association.14
Efferent nerve fibers originating from the hypothalamic preoptic sweat center descend through the ipsilateral brainstem and medulla and synapse in the intermediolateral cell columns of the spinal cord without crossing (although sympathetic vasomotor fibers may partially cross).15 The myelinated axons rising from the intermediolateral horn of the spinal cord (preganglionic fibers) pass out in the anterior roots to reach (through white ramus communicans) the sympathetic chain and synapse. Unmyelinated postganglionic sympathetic class C fibers arising from sympathetic ganglia join the major peripheral nerves and end around the sweat gland. The supply to the skin of the upper limb is commonly from T2 to T8. The face and the eyelids are supplied by T1 to T4, so that resection of T2 for the treatment of palmar hyperhidrosis is likely to cause Horner syndrome. The trunk is supplied by T4 to T12 and the lower limbs by T10 to L2. Unlike the sensory innervation, a significant overlap of innervation occurs in the sympathetic dermatome because a single preganglionic fiber can synapse with several postganglionic fibers.
The major neurotransmitter released from the periglandular nerve endings is acetylcholine (Ach), an exception to the general rule of sympathetic innervation, in which noradrenaline is the peripheral neurotransmitter. In addition to ACh, adenosine triphosphate (ATP), catecholamine, vasoactive intestinal peptide, atrial natriuretic peptide, calcitonin gene-related peptide, and galanin have been localized in the periglandular nerves. The significance of these peptides or neurotransmitters in relation to sweat gland function is not fully understood.
Botulinum toxin interferes with ACh release. Its heavy chain binds the neurotoxin selectively to the cholinergic terminal and the light chain acts within the cells to prevent ACh release. Type A toxin cleaves sensory nerve action potential-25, a 25-kDa synaptosomal-associated protein; the type B light chain cleaves vesicle-associated membrane protein (also called synaptobrevin). Botulinum toxins are used for symptomatic relief of hyperhidrosis.16 A more detailed description can be found in Chapters 84 and 255.
In humans, the sweating response to intradermal injection of nicotine or ACh disappears within a few weeks after denervation of the postganglionic fibers,17 while the sweating response to heat ceases immediately after resection of the nerves. In contrast, after denervation of preganglionic fibers (due to spinal cord injuries or neuropathies), pharmacologic responsiveness of the sweat glands is maintained from several months to 2 years, even though their thermally induced sweating is no longer present.18
Sweating induced by emotional stress (emotional sweating) can occur over the whole skin surface in some individuals, but it is usually confined to the palms, soles, axillae, and the forehead. Emotional sweating on the palms and soles ceases during sleep, whereas thermal sweating occurs even during sleep if the body temperature rises. Because both types of sweating can be inhibited by atropine, emotional sweating is cholinergically medicated.
Sweat glands respond to cholinergic agents, α- and β-adrenergic stimulants, and other periglandular neurotransmitters, such as vasoactive intestinal peptide and ATP. Periglandular ACh is the major stimulant of sweat secretion, and its periglandular concentration determines the sweat rate in humans. When dissociated clear cells are stimulated in vitro by cholinergic agents, they lose K+ & Cl−, increase intracellular Ca2+, and shrink, mimicking actions seen in vivo.19 Striking individual differences exist in the degree of sweating in response to a given thermal or physical stress. In general, males perspire more profusely than females.20 The sweat rate in a given area of the skin is determined by the number of active glands and the average sweat rate per gland. The maximal sweat rate per gland varies from 2 to 20 nL/min2. Sweat rate increases during acclimatization, but the morphologic and pharmacologic bases of the individual and regional differences in sweating rate during acclimatization are still poorly understood (Fig. 83-4). In thermally induced sweating, the sweat rate can be mathematically related to the body and skin temperatures in a given subject only in the low sweat rate range. Cholinergic stimulation yields a five to ten times higher sweating rate than does β-adrenergic stimulation. α-Adrenergic stimulation (by phenylephrine) is no more potent than isoproterenol (ISO) (a β-adrenergic agonist) in humans in vivo.21 Whereas cholinergic sweating begins immediately on intradermal injection, β-adrenergic sweating requires a latent period of from 1 to 2 minutes, which suggests that the intracellular mechanism of sweat induction may be different for methacholine and for ISO. Because the sweat rate in response to adrenergic agents is rather low, it may be reasonable to surmise that adrenergic stimulation in periglandular nerves may be involved in the regulation of sweat gland function but not in the induction of sweat secretion. One consequence of dual cholinergic and adrenergic innervation is to maximize tissue accumulation of cyclic adenosine monophosphate, which may be instrumental in stimulating the synthesis of sweat and glandular hypertrophy of the sweat gland. The possibility that periglandular catecholamine is directly involved in emotional sweating or sweating associated with pheochromocytoma22 may be ruled out, because these sweating responses can be blocked by anticholinergic agents.
Figure 83-4
Individual variation in the size of the sweat gland in four male adults, aged 22–28 years. Sweat glands were isolated from skin biopsy specimens obtained from the upper back behind the axilla. Subject 1 is a sedentary man who does not exercise regularly, whereas subject 4 is a well-acclimatized athletic individual.
The periodicity of sweat secretion in vivo is caused by the periodicity of central nerve impulse discharges, which occur synchronously with vasomotor tonus waves. Myoepithelial contraction occurs with cholinergic stimulation, but neither α- nor β-adrenergic agents induce tubular contraction.23 While the myoepithelium may contribute to sweat production via pulsatile contractions, it also seems to provide structural support for the secretory epithelium, especially under conditions in which stagnation of sweat flow (due to ductal blockade) results in an increase in luminal hydrostatic pressure.8
Sweat secretion is mediated by the energy (i.e., ATP)-dependent active transport of ions, so a continuous supply of metabolic energy is mandatory for sustained sweat secretion. Endogenous glycogen stored in the clear cells can sustain sweat secretion for less than 10 minutes; thus the sweat gland must depend almost exclusively on exogenous substrates for its energy metabolism. Mannose, lactate, and pyruvate are used nearly as readily as glucose; other hexoses, fatty acids, ketone bodies, intermediates of the tricarboxylic acid cycle, and amino acids are either very poorly used or not used as substrates. The physiologic significance of lactate or pyruvate utilization by the sweat gland is not yet clear. However, because the plasma level of glucose (5.5 mM) is much higher than that of lactate (1–2 mM) or pyruvate (less than 1 mM), glucose may play a major role in sweat secretion. Oxidative metabolism of glucose is favored as the major route of ATP formation for secretory activity.23
Sweat is formed in two steps: (1) secretion of a primary fluid containing nearly isotonic NaCl concentrations by the secretory coil, and (2) reabsorption of NaCl from the primary fluid by the duct. Although a number of factors affect ductal NaCl absorption, the sweat rate (and thus the transit time of sweat) has the most important influence on final NaCl concentration. Sweat NaCl concentration increases with increasing sweat rate to plateau at around 100 mM (Fig. 83-5). Potassium (K+) concentration in sweat is relatively constant. It ranges from 5 to 10 mM, which is slightly higher than plasma K+
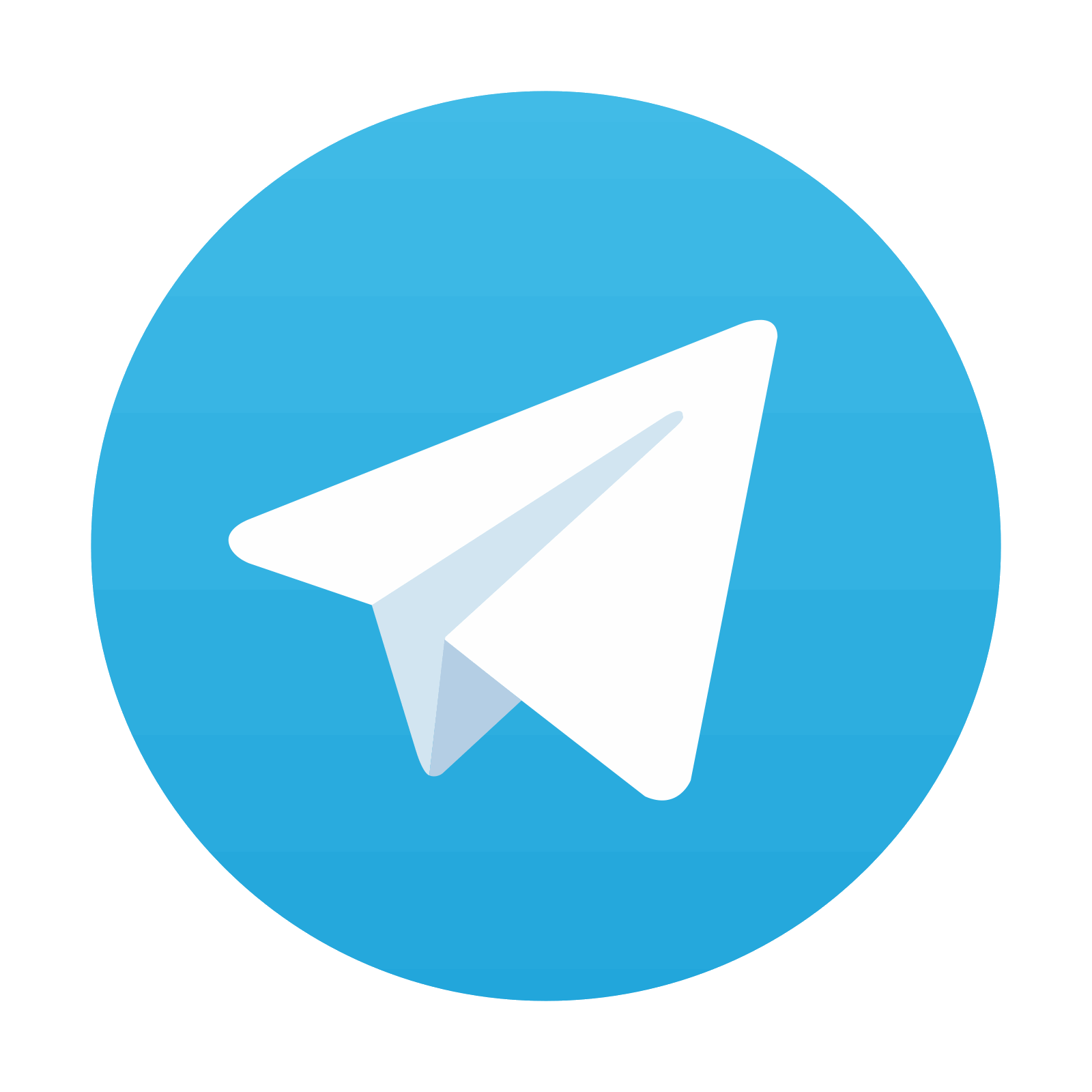
Stay updated, free articles. Join our Telegram channel
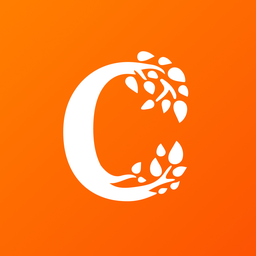
Full access? Get Clinical Tree
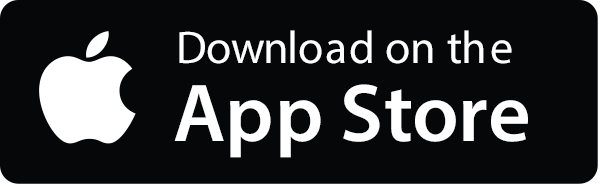
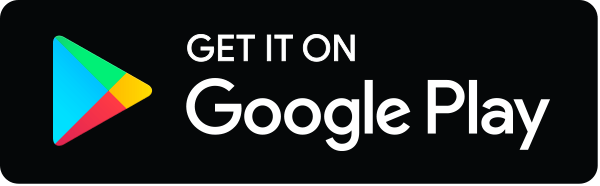
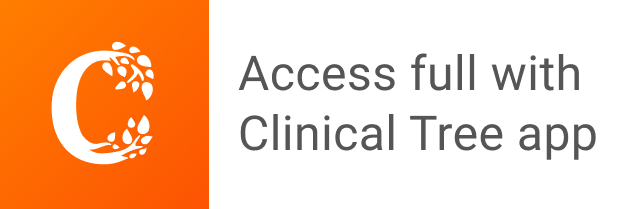