Fig. 8.1
Scheme of microcirculatory vessels network; A1 first-order arteriole, A2 second-order arterioles, A3 third-order arterioles (Documentation belongs to the author)
These considerations were quickly followed by designs of novel microcirculatory models, which were to provide objective evidence on the conditions and mechanisms of microcirculation during long-lasting surgical reconstructive procedures. This evidence could become a future means for improving surgical, anesthesiological, and pharmacological techniques, as well as monitoring of any physiological or pathological changes in microcirculation that could directly or indirectly affect microvascular function [21].
For years, various in vitro and in vivo models of microcirculation have been introduced in scientific research. Advances in technology and understanding of the physiological mechanisms of microcirculation have led to constructing a model that allowed for observation and assessment of microcirculation in vivo, with full visualization of anatomical structures and blood morphotic elements flowing through microcirculatory vessels or found outside microvascular space. The most well-known of these models include, the musculocutaneous flap in pigs, buccal flap model in hamsters, diaphragmatic muscle flap in rats, and the cremaster muscle in rats; the latter subsequently became the most universal of all experimental microcirculatory models [5, 15, 19, 20].
Thus, the cremaster model has been the most extensively described in literature. In 1964, Grant conducted the first observation of a specially exposed surface of the cremaster muscle. This observation irrefutably demonstrated that the cremaster in rats is a typical skeletal muscle in terms of its anatomical and histological structure, innervation type, and reaction to vasoactive substances, which makes this model unique [4]. Later, Siemionow was the first to dissect the rat cremaster muscle on its neurovascular pedicle up to the place where its arteries branch out from the external iliac artery and its veins connect with the external iliac vein. This marked the creation of a unique free muscular flap model allowing for its complete transposition out of the animal’s body to observe microcirculation via an in vivo microscopic technique. A photograph of a cremaster muscle flap model in presented in Fig. 8.2.
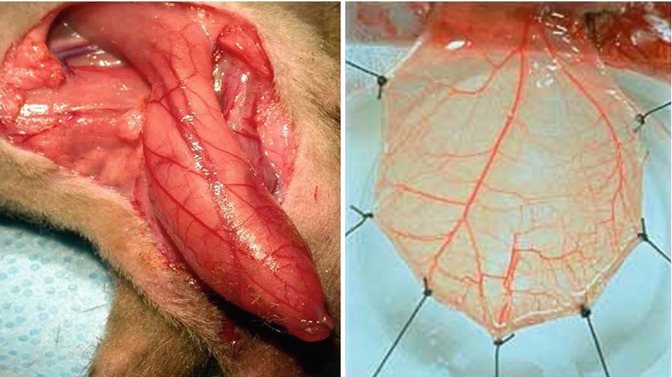
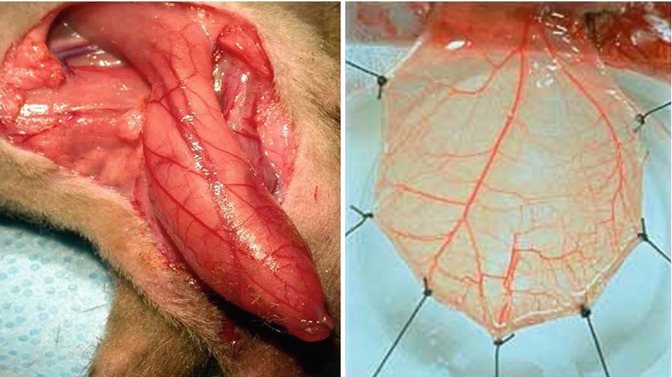
Fig. 8.2
Cremaster muscle flap model (Documentation belongs to the author)
Moreover, this model allowed for a complete denervation of the free muscular flap, which has been essential for differentiation between the effects of native neurological regulation of muscular vasculature and the effects of medications, anesthetic agents, surgical techniques, acid-base balance, and oxygen supply in an experimental setting, which often reflects the phenomenon of ischemia and reperfusion, inexorably related to morbidity and mortality rates [5, 14, 22–24, 30].
Thus, the question arises whether the conditions for analysis of microcirculatory phenomena in various experimental models fully reflect the actual processes in a living organism that has not been subject to a surgical procedure and anesthesia? In order to understand this issue, the design and execution of an in vivo experimental model must be considered. One criticized limitation of some experimental models involves the selection of laboratory animals. For example, the necessity of using animals of low body weight (approximately 130–180 g) in the free muscular flap model in rats, as this is the only way the muscle can be subject to transilumination, with the level of transparency allowing for in vivo microscopic examination at maximum magnification of up to 1,800×. It also requires the use of surgical microscopy to dissect the free muscular flap itself, as well as cannulation of arterial and venous vessels to monitor hemodynamic parameters.
Another issue worth considering is the effect of invasive monitoring techniques (cannulation of major vessels, mechanical ventilation, intravenous fluid therapy) on changes in microcirculatory hemodynamics, since all these invasive aspects of the animal model are required for standardization purposes. These invasive techniques provide general stabilization of homeostasis via adequate oxygen supply and carbon dioxide elimination, which ensures physiological acid-base and electrolyte equilibrium due to intravenous fluid therapy and regulation of anesthesia depth, both adequately adjusted to the monitored hemodynamic parameters [5, 7, 8, 30]. Arranged in the manner described above, this experimental model ensures its reproducibility in terms of all measurable parameters, which increases the likelihood of it being used by researchers initiating experimental studies.
In order to objectively evaluate the changes in microcirculation in response to varying experimental conditions, certain objectively measurable parameters had to be identified, defined, and introduced. These include: vessel diameter (in consecutive arteriolar branch levels and in post-capillary venule), red blood cell velocity (in arterioles and post-capillary venule), leukocyte-endothelium interactions in post-capillary venule (rolling, adhering, and transmigrating leukocytes). Figure 8.3 presents a photograph of blood morphotic elements in a postacapillary venule.
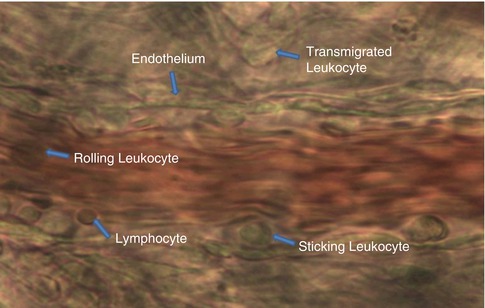
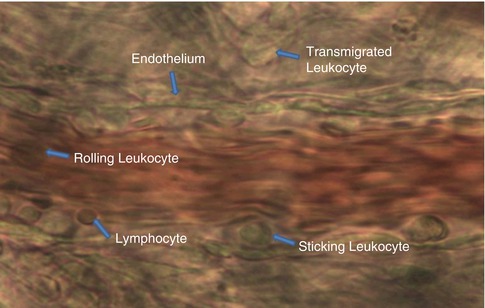
Fig. 8.3
Photograph of blood morphotic elements in postacapillary venule (magnification 1,800×) (Documentation belongs to the author)
Another evaluated parameter is endothelial edema index (calculated as the ratio of the external and internal post-capillary venule diameter), which objectively shows changes in vessel diameter during the time of the experiment. Another parameter assessed during microcirculation analysis is capillary perfusion (reflecting the number of capillaries in which the flow of blood morphotic elements could be seen).
The above criteria have helped standardize and objectively evaluate microcirculation assessments, irrespective of the experimental model selected for the given study [5, 7, 8, 18, 22, 30]. Therefore, as a result of years of microcirculatory research, the established set of data required for evaluating microvascular hemodynamics includes: the structures comprising arterial, capillary, and venous vasculature of the area together with characterization of the blood morphotic elements involved and perfusion velocity or volume depending on the measuring approach used. An example device for measuring microvascular blood perfusion may be optical Doppler velocimeter method for imaging red blood cell flow velocities coupled with intravital microscopy, or a laser Doppler flowmeter. Scheme of intravital microscopic measurments is presented in Fig. 8.4.
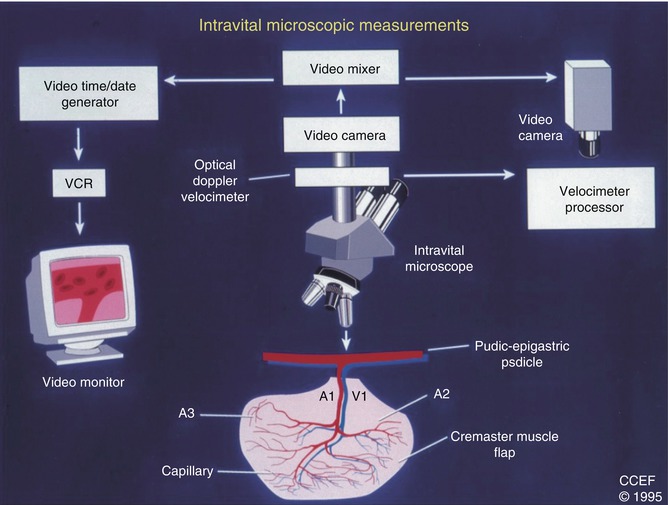
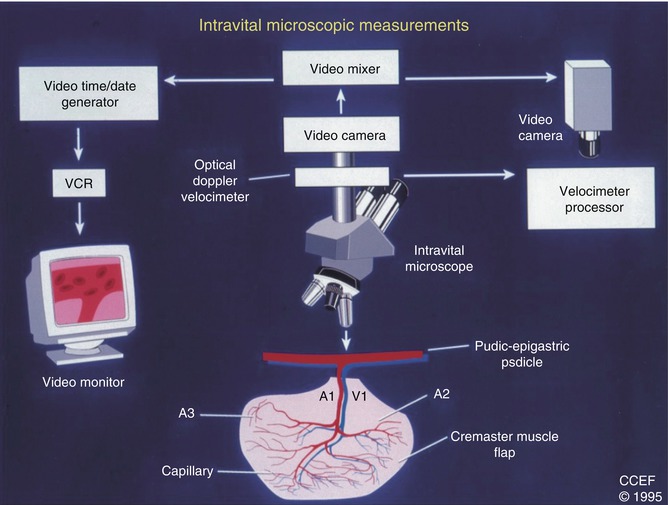
Fig. 8.4
Scheme of intravital microscopic measurements (Documentation belongs to the author)
Microsurgical and reconstructive procedures involving free tissue transfer often last many hours, with their duration usually exceeding the mean duration of a standard surgical procedure [6]. Thus, certain cardiovascular parameters must be obligatorily monitored during such long procedures, via methods appropriate in terms of the extent of the procedure and general condition of the patient, in order to avoid intraoperative complications and reduce intraoperative and postoperative morbidity and mortality. Direct monitoring of fundamental hemodynamic parameters such as mean arterial pressure (MAP), central venous pressure (CVP), mean pulmonary arterial pressure (MPAP), and rarely pulmonary capillary wedge pressure (PCWP), etc. requires the use of special catheterization techniques in selected vessels and often also body cavities, which is invasive in its nature [7]. A question arises as to the extent to which direct monitoring-oriented major vessel catheterization affects the established aspects of microcirculation, including the measured hemodynamic parameters themselves.
Nalbantoglu et al. demonstrated that the presence of multiple intravascular catheters, inserted to measure hemodynamic parameters (e.g. MAP, CVP) and collecting blood samples for arterial blood gas analyses (including pH, arterial partial oxygen pressure [PaO2], arterial partial carbon dioxide pressure [PaCO2]), constitutes a separate factor significantly affecting microvascular hemodynamics. This conclusion was based on observations in a group of rats that had their hemodynamic parameters measured invasively, where both the arteriole diameter and the number of perfused capillaries in the cremaster muscle flap were significantly reduced in comparison with those in a group of rats whose hemodynamic parameters were not monitored this way [8]. This phenomenon may be attributed to catheter-induced mechanical injury to the vascular endothelium and the subsequent microemboli formation, platelet activation, and increased leukocyte activity. Anderson et al. suggested that mechanical obstruction of vascular lumen is only one of the potentially responsible factors, since these changes also lead to endothelium-mediated vasoconstriction, which becomes another cause of microemboli formation [9].
Moreover, any mechanical manipulation associated with isolating the cremaster muscle flap can be argued to have a significant impact on local microcirculation. However, interestingly vascular cannulation in locations distant from the cremaster muscle flap, also had a statistically significant impact on reduced capillary perfusion, increased leukocyte activation (leukocyte rolling in the post-capillary venule) in comparison with those parameters in the group of animals not subjected to vessel cannulation, which proved the development of microcirculatory signs and consequences of a cannulation-induced systemic inflammatory reaction [7].
Moreover, we demonstrated the effects of intravascular cannulation in the form of increased red blood cell velocity, dramatically increased number of rolling leukocytes in post-capillary venule, increased number of leukocytes adhering to the endothelium along with increasing endothelial edema, which led to reduced vascular lumina and post-capillary venous return [8]. There is a fundamental discrepancy between the role of leukocytes in physiological and experimentally generated settings. Leukocyte activity depends considerably on the type of tissue to which they migrate, due to various capillary patterns in those tissues [10]. In physiological conditions, the number of leukocytes occurring spontaneously in post-capillary venule constitutes a negligible portion of the systemic leukocyte count and that portion has no effect on vascular resistance in muscles or in any other tissue. However, a significant increase in vascular resistance in the local microcirculation has been observed when the number of endothelium-associated leukocytes increased along with an increase in white blood cell count in the evaluated tissue, which may considerably contribute to dysfunction of the transposed or isolated muscular flap [7].
The techniques for hemodynamic parameter monitoring are essential for maintaining systemic homeostasis. Nonetheless, we should be aware of their negative subclinical effects on microcirculation. This constitutes a spectacular proof of the benefits of using an experimental model with vascular cannulation to visualize its negative effects on microcirculation itself. The resulting clinical implications of this observation are obvious.
Tissue exposure to various types of injuries, such as high temperature, surgical incision, tissue compression with the subsequent reduction in perfusion, also causes locally increased leukocyte activation and adhesion to vascular endothelium [10]. A given injury results in an increased vascular wall permeability and tissue edema, as well as formation of micro-emboli responsible for arteriole and venule obstruction.
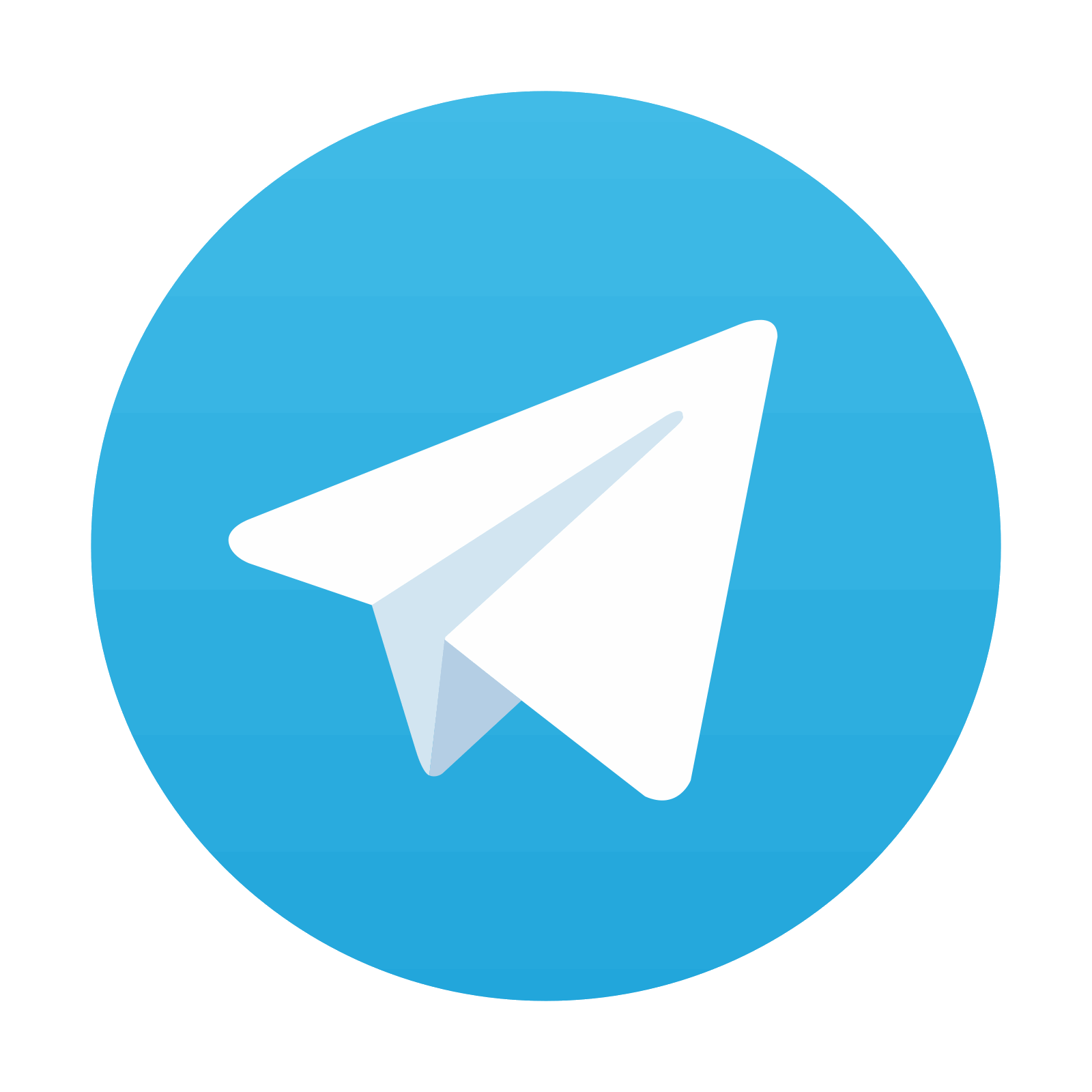
Stay updated, free articles. Join our Telegram channel
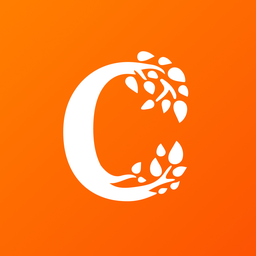
Full access? Get Clinical Tree
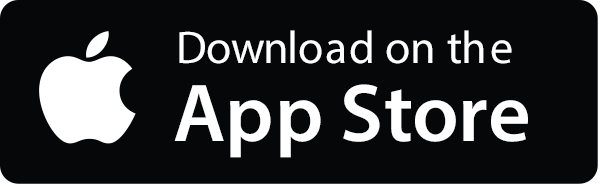
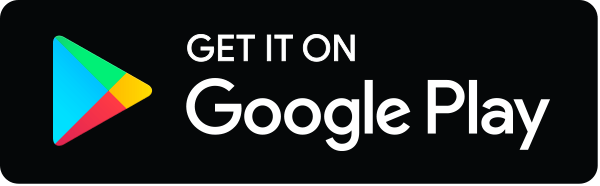