Fig. 12.1
Example of EPR spectra of a covalently bound drug/spin probe and of one released in solution
12.2 Nanocarriers
As well as penetration enhancers like ethanol and dimethylsulfoxide, various nano- and micrometer scaled carrier systems are used in pharmaceutics and cosmetics for enhanced skin penetration. Liposomes were the first nanosized drug carriers for the topical route of administration of triamcinolone, being the first drug liposomally encapsulated and applied to the skin of rabbits (Mezei and Gulasekharam 1980). Furthermore, microemulsions and nanoemulsions are used as drug carriers as well as lipid based nanoparticles. Polymer-based macromolecules, for example, dendrimers appear to be also promising nanocarriers.
In the following paragraphs, a selection of nanocarriers for dermal application is described in more detail.
12.2.1 Invasomes
Invasomes are highly fluidic, ultra-deformable, elastic liposomal vesicles, which consist of phosphatidylcholine, ethanol, and terpenes (Dragicevic-Curic et al. 2008). Due to their fluidic membrane, liquid-state vesicles exhibit superior drug penetration enhancement ability compared to conventional, rigid gel-state liposomes (El Maghraby et al. 2001). Liposomes are of spherical shape, whereas invasomes are often of deformed spherical shape due to their highly fluidic and deformable membrane. Visualization of invasomes revealed that they appear unilamellar and bilamellar, which is independent of the loaded agent (Chen et al. 2011).
Moreover, it could be shown that the combination of ethanol and terpenes can enhance skin penetration of drugs, e.g., the highly lipophilic drug midazolam (Ota et al. 2003), as well as the water-soluble diclofenac sodium (Obata et al. 1990, 1991). Therefore, invasomes promote the penetration of drugs with unfavorable partition coefficient (logP value) and molecular weight (MW), e.g., the lipophilic drug temoporfin (MW = 681 g/mol, logP = 9.3) (Dragicevic-Curic et al. 2008, 2009; Chen et al. 2011) and the hydrophilic model agent carboxyfluorescein (MW = 378 g/mol, logP = −1.5) (Chen et al. 2011). Two mechanisms are described in the literature, which explain how the interaction of elastic vesicles with the skin appears to enhance penetration. The first mechanism proposes that vesicles or vesicle constituents disorganize and disrupt intercellular lipids, affecting the ultrastructure of the stratum corneum by the creation or modification of pathways for possible drug penetration, thus leading to increased skin permeability. However, treatment with rigid vesicles did not affect stratum corneum ultrastructure or permeability (van den Bergh et al. 1999). The second mechanism postulates that ultraflexible vesicles can penetrate the skin unfragmented on areas with low penetration resistance, e.g., between neighboring corneocyte clusters with no lateral overlapping of the corneocytes (Schätzlein and Cevc 1998; Cevc et al. 2002). Moreover, it was found that ultraflexible vesicles penetrate intact into deeper stratum corneum layers; however, only small amounts were found in the deepest stratum corneum layers. These findings could not be observed after the application of rigid gel-state vesicles (Honeywell-Nguyen et al. 2002).
12.2.2 Core Multishell Nanotransporters
Core multishell (CMS) nanotransporters are chemical chameleons, which, simular to liposomes, can encapsulate both hydrophilic and lipophilic guest molecules. Additionally, they can be dissolved in a variety of solvents, ranging from water to toluene. The unimolecular architecture of CMS nanotransporters contains a hydrophilic core, followed by a hydrophobic middle layer, and a hydrophilic outer shell. This architecture mimics the structure of liposomes, which are lipid vesicles that comprise of an aqueous inner phase, enclosed by a phospholipid membrane and surrounded by an exterior aqueous phase. As described above, the liposome architecture was found to have a penetration enhancing effect. It has been suggested that liposomes adhere to the skin surface and destabilize, fuse, or mix with the lipid matrix, resulting in a loosening of the lipid structure, which lowers the barrier strength of the skin and enhances skin penetration of loaded drugs or agents (Elsayed et al. 2007; Kirjavainen et al. 1996). Due to their liposome-like architecture, CMS nanotransporters might exhibit a similar penetration enhancing efficiency and additionally, as polymer-based nanotransporters, an improved stability.
The terminal shell provides a good solubility of the particle in water as well as in organic solvents and a high degree of biocompatibility. Furthermore, as well as the core, it serves as a matrix for encapsulation of hydrophilic agents. The nonpolar inner shell allows incorporation of hydrophobic guest molecules (Radowski et al. 2007).
CMS nanotransporters comprise of small unimers, 5 nm in size, but if the critical aggregation concentration of 0.1 g/l is reached, larger aggregates are formed with a diameter of approximately 30–50 nm. β-Carotene loaded CMS nanotransporters form aggregates up to 144 nm in size (Radowski et al. 2007).
CMS nanotransporters were shown to enhance skin penetration of the lipophilic dye Nile red (Küchler et al. 2009b) and the hydrophilic dye rhodamine B (Küchler et al. 2009a) and thus appear to be of outstanding versatility. Furthermore, CMS nanotransporters are used for tumor targeting (Quadir et al. 2008), cellular copper uptake by yeast cells (Treiber et al. 2009), or as stabilizers for catalytic platinum nanoparticles (Keilitz et al. 2010).
12.2.3 Nanostructured Lipid Carriers
Nanostructured lipid carriers (NLCs) consist of a solid lipid matrix formed by, e.g., glycerol tristearate (Dynasan® 118; Cognis, Monheim, Germany) and are loaded with liquid lipids of, e.g., caprylic/capric triglycerides (medium chain triglycerides, Miglyol® 812, Caelo, Hilden, Germany). NLCs are dispersed in water and stabilized by an emulsifying agent. However, the structure of NLCs is described differently in the literature. One theory describes NLCs as spherical nanoparticles with droplets of liquid lipid within the solid lipid matrix (Müller et al. 2002), whereas another group describes them as lipid platelets with oil spots located on the surface of the solid lipid matrix (Jores et al. 2004). Preparation of NLCs is performed by high-pressure homogenization (HPH), applying two to three cycles at 500 bar. HPH can be performed at high temperatures, e.g., 80 °C but also cold HPH is known (Müller et al. 2000). HPH at room temperature is particularly interesting for the loading of temperature-sensitive agents to NLCs. The disadvantage of cold HPH is the increase in particle size. Mean particle size exhibits a wide range from 50 to 1000 nm. The lipid content of a formulation/nanodispersion can be from 5 to 40 % (Schäfer-Korting et al. 2007). NLCs were used for the controlled release of the highly lipophilic drug clotrimazole (Souto et al. 2004). Moreover, topical application of NLCs results in the formation of an occlusive film on the skin which improves skin hydration (Müller et al. 2002). Nevertheless, NLCs failed to enhance the uptake of Nile red (Lombardi Borgia et al. 2005) and cyproterone acetate (Stecova et al. 2007) when compared to the uptake from solid lipid nanoparticles.
12.3 Electron Paramagnetic Resonance Spectroscopy
EPR is a powerful tool for the detection of free radicals and other paramagnetic molecules (molecules with one or more free, unpaired electrons in the outer orbital shell). The concept of EPR spectroscopy is similar to nuclear magnetic resonance (NMR) spectroscopy. Both are based on the interaction of electromagnetic radiation with magnetic moments. In EPR spectroscopy the magnetic moment originates from free unpaired electrons of a molecule, whereas in NMR spectroscopy the magnetic moment arises from the proton spin of hydrogen nuclei.
Therefore, EPR spectroscopy is concerned with resonant absorption of microwave energy by an unpaired free electron within an applied magnetic field. The energy of a free electron in a magnetic field splits into two distinct energy levels, which is called the Zeeman effect (Fig. 12.2). If the energy of the microwave radiation corresponds to the energy difference of the two distinct energy levels of an electron in a magnetic field, resonance conditions are fulfilled and spin conversion occurs. This results in a change of microwave energy, which is detected in EPR spectroscopy.


Fig. 12.2
Zeeman effect. The magnetic field splits the energy of a free electron into two distinct energy levels. Resonance conditions are fulfilled if the microwave energy fits the energy difference between the two energy levels
The fundamental equation of EPR spectroscopy is given in formula Eq. 12.1:

with h as the Planck constant, ν the microwave frequency, g the Landé g-factor, μ B the Bohr magneton, and B 0 the magnetic field strength.

(12.1)
EPR spectrometers are classified by the microwave frequency, which ranges from 0.3 GHz (Alecci et al. 1994) to 360 GHz (Fuchs et al. 2002). L-band EPR spectrometers operate at a frequency of 1.3 GHz, X-band at 9.4 GHz, Q-band at 34 GHz, and W-band at 94 GHz, and the frequency determines the measuring sensitivity of an EPR spectrometer. Sensitivity is directly related to the square of operating frequency (Fuchs et al. 2001).
Moreover, the dependency of microwave frequency with microwave penetration into biological tissue is of high importance: At L-band frequency, microwave penetration amounts to 20 mm and is reduced exponentially at X-band frequency to below 1 mm (Fuchs et al. 2001). This can be explained by the high water content of biological samples, which causes high nonresonant, dielectric absorption of microwaves. Nonresonant absorption is a function of frequency and increases with increasing frequency.
Besides detection and quantification of nitroxides or other paramagnetic molecules, EPR spectroscopy can provide information on the physicochemical properties of the molecule as well as information about its immediate surrounding, i.e., polarity and viscosity. This information can be derived from the line shape of the EPR spectrum. EPR spectra of nitroxides in solution comprise of three resonant lines (Fig. 12.3), which are due to the magnetic moment of the neighboring nitrogen nucleus. Interactions of free, unpaired electrons with the magnetic moment of the nitrogen nucleus are described as 14N hyperfine coupling, which splits the EPR spectrum in three lines. The 14N hyperfine coupling constant (aiso) is strongly influenced by hydrogen bonds between solvent molecules and the oxygen of the nitroxide and therefore, gives information about the polarity of the immediate surrounding of the paramagnetic molecule. It was found that aiso increases proportionally with the concentration of hydrogen donor groups of the solvent (Gagua et al. 1978).


Fig. 12.3
EPR spectrum of the nitroxide PCA that illustrates typical EPR parameters: I: signal amplitude, aiso: hyperfine coupling constant, lw: peak to peak line width
The g-factor indicates the position of the resonant lines within the magnetic field. Moreover, it also reflects the polarity of its immediate surrounding when measuring at high frequencies as shown in Fig. 12.4 for Q- and W-band measurements. The g-factor increases with increasing lipophilicity of the microenvironment. A further indicator of the polarity is the hyperfine coupling constant, aiso, which reflects the polarity independent of the applied frequency. Low values of aiso represent a lipophilic environment, whereas high values a hydrophilic one, e.g., the nitroxide 2,2,6,6-tetramethyl-1-piperidinyloxy (TEMPO; Sigma Aldrich, Steinheim, Germany) has an aiso of 1.74 mT in water and 1.59 mT in a mix of phosphatidylcholine and lysophosphatidylcholine (Haag et al. 2011c).


Fig. 12.4
Chemical structure of selected nitroxide spin probes: TEMPO (left) and PCA (right)
An indicator of the microviscosity is the rotational correlation time in seconds, which is defined as the time it takes for the spin probe to rotate once around its own axis. This is one of the main features of EPR spectroscopy when studying drug release processes, e.g., a spin probe or spin labeled drug that is incorporated in a carrier system can be differentiated from a released one, especially if it is covalently bound to a nanoparticle (see spectra in Fig. 12.1).
Additionally, in the case of highly concentrated paramagnetic substances within carrier systems a broadening of the signals appears due to spin–spin coupling. In the case of release, the broadening disappears.
The four described EPR parameters can be derived from the EPR spectrum by using simulation software, e.g., the EasySpin toolbox (Stoll and Schweiger 2006).
12.4 Stable Nitroxide Spin Probes—Aminoxyl Radicals
Nitroxides are versatile spin probes for various applications in biology, medicine, and pharmaceutics. Nitroxides are paramagnetic species, possessing a free, unpaired electron in the outer shell of the molecule and are therefore detectable by EPR spectroscopy. While all have a free electron at the nitrogen in common, the chemical structures and thus physicochemical properties can differ significantly. Most commonly applied nitroxides are six-ring systems (piperidines) and five-ring systems (pyrrolidines). The stability of the free radical arises from the delocalized electron from the NO bond and steric hindrance by the four methyl groups.
Two representative spin probes are 3-carboxy-2,2,5,5-tetramethyl-1-pyrrolidinyloxy (PCA; Sigma Aldrich, Steinheim, Germany), (logP = −1.7; MW = 186 g/mol) and TEMPO (logP = 2.3; MW = 156 g/mol) (Fig. 12.4). The stability of the nitroxide is mainly determined by its ring structure, PCA with its five-ring system is significantly more stable than TEMPO with its six-ring system (Fuchs et al. 1993). Both spin probes can be applied in vivo (Fuchs et al. 1997).
12.5 Applications
12.5.1 Partitioning of a Drug Within a Carrier
EPR spectroscopy allows distinguishing between environments differing in polarity (Kempe et al. 2010). Smirnov et al. (1995) could show TEMPO partitioning between the aqueous and lipid domains of liposomes. Previously, Haag et al. (2011a) studied ultraflexible liposomes (invasomes) and nanostructured lipid carriers, which had also been prepared with TEMPO. Partitioning of TEMPO between lipid and aqueous phases could be observed and quantified. Changes in partitioning after application to the skin resulted in a sustained release of TEMPO to the skin. In Fig. 12.5, EPR spectra of TEMPO-loaded invasomes (solid, black line) recorded at different microwave frequencies are shown. Sensitivity is directly related to the square of operating frequency, which means the higher the frequency, the higher the measuring sensitivity of the spectrometer. Due to the low magnetic field strength at L-band frequency (1.3 GHz), spectra from phases originating from different polar environments appear as a single three-line spectrum, because the spectra from the different environments overlap. By increasing the frequency to X- and Q-band, spectra from the different phases are separated more efficiently. Measurements at W-band frequency (94 GHz) can completely separate the spectra from environments differing in polarity due to the high magnetic field strength, which results in two spectra. In this case one from the phosphatidylcholine/lysophosphatidylcholine phase and one from the aqueous phase of the invasomes. Since we can separate the spectra at W-band, the magnetic parameters can be fully derived from the spectra in each phase (i.e., g-value indicates the position of the spectra within the magnetic field), 14N hyperfine coupling (aiso, distance between low field and central line, high values indicate a hydrophilic environment and vice versa), and the rotational correlation time (the time it takes for a spin probe to turn once around its own axis). This is a prerequisite for spectral simulation of spectra recorded at lower frequencies. By entering these parameters into computer software, e.g., EasySpin the spectra can be simulated at each frequency as shown in Fig. 12.5 (gray, dashed line).


Fig. 12.5
Multifrequency measurement of the spin probe TEMPO within an invasome dispersion. The asterisk indicates signals of TEMPO from the lipophilic membrane and the arrows from the aqueous phase
The L-band low frequency measurement shows a broadened low and high-field line. The spectrum at X-band frequency shows a partitioned high-field line. When measuring at Q-band frequency, two lines of the lipophilic TEMPO EPR spectrum already appear and are shifted down-field due to higher g-values. The measurement at W-band clearly resolves three lines from each of the two phases. As demonstrated in Fig. 12.5, the resolution increases with increasing frequency. At W-band frequency the distribution between phases of different polarity can be determined, and most importantly quantified. This is especially important for the development of lipid-based carrier systems for a specific purpose, e.g., in the surfactant shell of the nanoparticle for burst release or in the core of a liquid lipid emulsion for sustained release. At W-band frequency, the distribution can be easily determined and quantified..
The use of different spectrometers operating at diverse microwave frequencies allows comprehensive studies of pharmaceutical formulations. At high frequency W-band (94 GHz) the localization of a spin probe or a spin-labeled drug within a carrier matrix becomes feasible allowing the observation of drug–carrier interactions. This can be the localization in a certain polar environment in a two-phase system like invasomes, or the localization of a spin probe in the core of a lipid nanoparticle or within the surfactant shell. Especially interesting for the investigation of topical dermatics is the low frequency L-band (1.3 GHz) EPR spectroscopy, because measurements of formulations containing spin probes applied to the skin ex vivo and in vivo are feasible. This allows the determination of penetration efficiency after skin application of spin probe loaded carriers. Moreover, it can be determined whether a carrier system releases its payload slowly in a depot manner.
Measurements to investigate the stability of delivery systems were further performed by Yucel et al. (2012), who investigated the distribution of the hydrophobic nitroxide 4-phenyl-2,2,5,5-tetramethyl-3-imidazoline-1-oxyl (PTMIO). PTMIO was homogenized with eicosane and a sodium caseinate solution. After cooling, solid lipid nanoparticles were formed, because eicosane is crystalline at 21.5 °C. Moreover, a further emulsion-based delivery system with tetradecane as the lipid phase was produced the same way. The only difference is that tetradecane forms liquid droplets at 21.5 °C. For both delivery systems, two different sizes were produced, fine droplets with a diameter of 0.2 μm and coarse droplets with 1.3 μm in diameter.. The distribution of the probe can be obtained from analysis of the spectra, and the chemical stability by measurements of reduction kinetics (e.g., vitamin C, which turns PTMIO EPR silent) as demonstrated in Fig. 12.9 where the degradation of TEMPO by the antioxidant system of the skin is shown. Analysis of the resulting EPR spectra revealed populations of the spin probe in two discrete environments (i.e., aqueous and lipid). PTMIO is largely hydrophobic with 77 and 70 % present in the coarse and the fine liquid lipid droplets of tetradecane, respectively. In the solid droplets (i.e., eicosane droplets) the probe was completely excluded from the droplets into the aqueous environment. Yucel et al. (2012) presumed that during the crystallization process the probe moves out of the solid lipid to the aqueous environment where it is more affected by the presence of the aqueous portions of the interfacial protein (sodium caseinate solution). Braem et al. (2007) showed that the EPR active cholestane is only located in the surfactant shell of solid lipid nanoparticles but not in the solid lipid core.
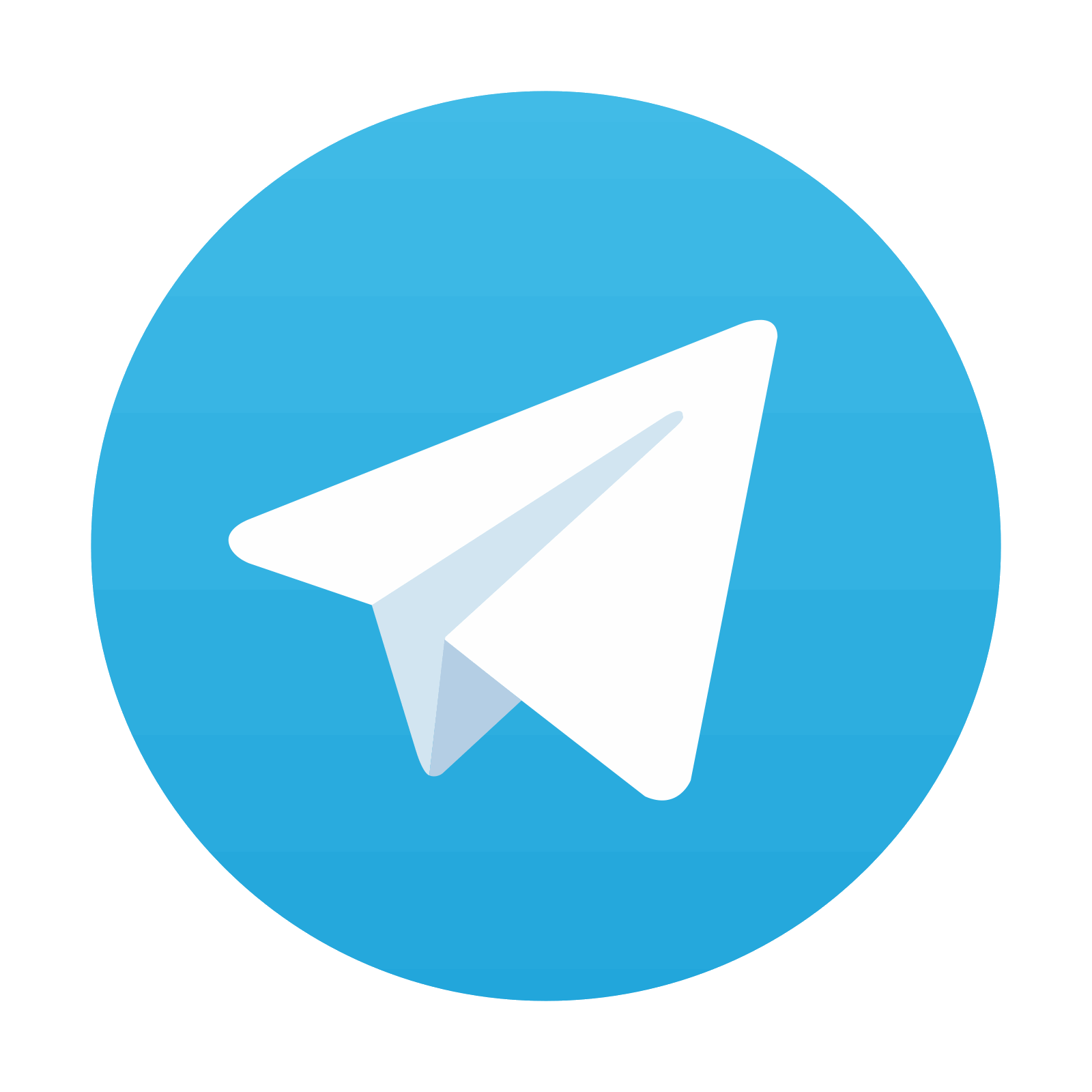
Stay updated, free articles. Join our Telegram channel
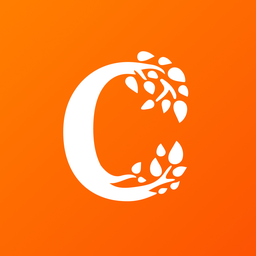
Full access? Get Clinical Tree
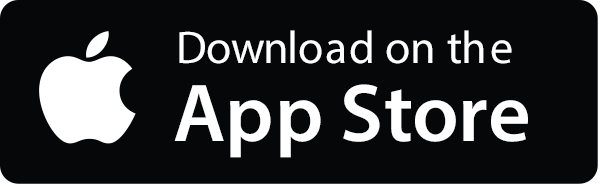
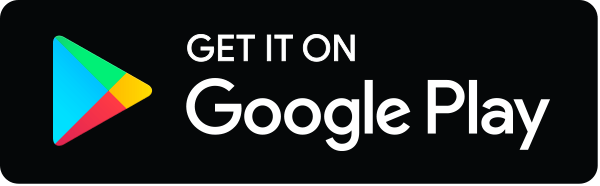