1
Anatomy, Embryology, and Normal and Abnormal Development of the Craniovertebral Junction and Cervical Spine
The craniovertebral junction (CVJ) and cervical spine are among the most complex areas of the spine, both anatomically and embryologically. Because these regions develop from several different embryonic components, the opportunities for anatomical variants and embryological malformations in this region abound. A working knowledge of the anatomy, embryology, and many abnormal variants involving the CVJ and cervical spine is crucial to properly identifying the congenital nature of these abnormalities and differentiating them from traumatic lesions, as well as navigating the surgical approaches to this region. This chapter reviews the normal anatomy of the bony and ligamentous structures of the CVJ and cervical spine, the normal developmental sequence that results in these complex structures, and the embryopathological substrate upon which the various developmental malformations in this region can be organized.
The chapter presents topics regarding the subaxial cervical spine before topics regarding the CVJ. Convention usually dictates that the more cranial anatomical elements are presented first. As the reader will become aware, however, the embryological theories regarding CVJ development are built upon the theories of subaxial cervical spine development, and therefore, the lower cervical anatomical elements are presented first in the interest of clarity.
Anatomy of the Subaxial Cervical Spine
Bony, Disk, and Ligamentous Anatomy
The third to sixth cervical vertebrae are regarded as typical vertebrae, and the seventh cervical vertebra is a transitional vertebra. Each of the vertebrae from C3 to C6 has a small, broad body and a large, triangular vertebral foramen. The spinous processes are short, are usually bifid, and may be palpable. At the junctions of the pedicles and lamina, each vertebra has a pillar that gives rise to the superior and inferior articular processes. These processes present horizontally oriented facets. The superior facets are directed upward and backward, and the inferior facets are directed downward and forward. From C3 to C6, each transverse process is pierced by a foramen transversarium. Small projections, or tubercles, emanate from the transverse processes.
The seventh cervical vertebra is a transitional vertebra because its long spinous process does not bifurcate but ends in a tubercle that gives attachment to the ligamentum nuchae. Its transverse process is large, and the foramen transversarium is small or even absent. Rarely, the vertebral artery passes through the foramen transversarium of C7. The costal process of C7 may develop separately and form a cervical rib.
The intervertebral disks are comprised of a central nucleus pulposus surrounded by the anulus fibrosus. The firm attachment of the intervertebral disks to the vertebral bodies constitutes a major supportive structure of the cervical spinal canal.
There are two main ligamentous attachments of the subaxial cervical spine. The anterior longitudinal ligament (ALL) runs along the anterior portion of the vertebral bodies and intervertebral disks, and the posterior longitudinal ligament (PLL) runs along the posterior portion of the same structures. They also are major supportive structures of the cervical spinal column. The superior extent of the ALL fuses to the anterior portion of the atlas, and the superior extent of the PLL fuses with the tectorial membrane. The ALL and the PLL both extend inferiorly to the sacrum.
The interspinous ligament, located between each spinous process from C3 to C7, is a minor supportive structure of the spinal column. The capsular ligaments surrounding the facet joints are also minor supportive structures.
Vascular and Lymphatic Anatomy
The most important vascular structure in the subaxial cervical spine is the vertebral artery. The vertebral artery courses through the soft tissues of the neck until it pierces the foramen transversarium at C6 and extends to the foramen transversarium of C3. The paraspinous tissue and vertebral column are fed by small vertebral artery branches.
The lymphatic drainage of the subaxial cervical spine is primarily into retropharyngeal lymph nodes and the cervical lymphatic chain.
Anatomy of the Craniovertebral Junction
Bony and Ligamentous Anatomy
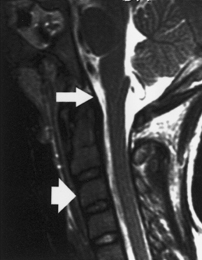
Figure 1–1 Anatomy of the craniocervical junction.
The bony portion of the CVJ is composed of the occipital bone, the atlas (C1), and the axis (C2) (Fig. 1–1, bony relationships). The occipital bone makes up the posterior membranous cranium as well as the inferior skull base. The foramen magnum is formed from a ring of bony structures derived from the occipital bone and includes the inferior clivus anteriorly, the occipital condyles laterally, and the opisthion posteriorly. The paired hypoglossal canals originate in the anterolateral portion of the clivus superior to the occipital condyles. The paired occipital condyles articulate with the superior surface of the atlas facets, forming the atlanto-occipital facet joint.
The atlas consists of a bony ring that interfaces between the occipital condyles superiorly and the axis inferiorly. The atlas lacks a vertebral body and instead has arches that project anteriorly and posteriorly from the paired atlanto-occipital facet joints. The atlas articulates superiorly with the occiput and inferiorly with the axis; the inferior articular facets project medially and posteriorly to articulate with the superior facets of the axis. The atlas thus serves as a “washer” between the occipital condyles above and the axis below. The atlas has small tubercles bilaterally along the inner surface of the anterior arch for the attachment of the transverse ligament. The anterolateral portion of the atlas contains atretic transverse processes that house the vertebral foramina. A groove within the superior surface of the posterior arch of the atlas is present for the vertebral artery.
The tectorial membrane, an important stabilizing structure of the atlanto-occipital joint, inserts into the anterior–inferior clivus and continues inferiorly as the PLL [Fig. 1–2, midsagittal magnetic resonance imaging (MRI)]. Other ligaments include the atlanto-occipital membrane, located in the dorsal aspect of the spinal canal, and the atlanto-occipital joint capsule supports. The atlantoaxial joint capsule articulations are lax and are reinforced laterally by the atlanto-occipital ligaments. The transverse ligament works as a check ligament to keep the odontoid snugly in place against the anterior arch of the atlas. Other minor ligaments that provide attachment of the axis to the occiput include the alar, apical dens, and cruciform ligaments.
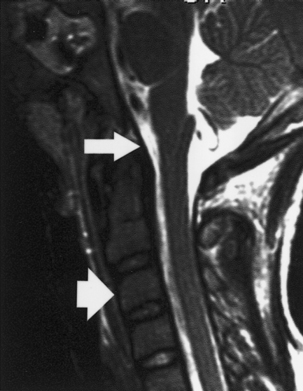
Figure 1–2 Sagittal magnetic resonance imaging showing the posterior longitudinal ligament (arrow) and the anterior longitudinal ligament (arrowhead).
Vascular and Lymphatic Anatomy
The vascular supply of the CVJ comes from branches directly off the vertebral artery and from occipital branches of the carotid artery. A vascular arcade is formed around the odontoid process, but the embryological origin of the arcade precludes vascular communication between the base of the dens and the body of the axis. This anatomical relationship helps explain the origin of os odontoideum (OO) and the frequent nonunion of type II odontoid fractures.
The lymphatic drainage of the CVJ is primarily into retropharyngeal lymph nodes and the deep upper cervical lymphatic chain. The venous plexus around the odontoid drains into the suboccipital epidural sinuses and appears to have a direct communication with the pharyngovertebral veins. Pharyngovertebral– lymphovenous anastomoses have been described.1 These communications are important in the genesis of Grisel’s syndrome, an inflammatory or infectious retropharyngeal condition causing atlantoaxial rotatory subluxation.
Embryology of the Cervical Spine
The normal development of the vertebral column includes six separate but overlapping phases: (1) gastrulation and the formation of the somitic mesoderm and notochord; (2) condensation of the somitic mesoderm to form the somites; (3) reorganization of the somites to form dermomyotome and sclerotome; (4) the membranous phase of somitic development and resegmentation of the somites to form the definitive vertebrae; (5) vertebral chondrification; and (6) vertebral ossification.
Gastrulation and Formation of Somites and Notochord
During the first 2 weeks following fertilization, the human embryo undergoes several cell divisions and cellular rearrangements that ultimately form a blastocyst, a two-layered embryo suspended between the amnionic and yolk sacs (Fig. 1–3). Cells on the dorsal surface of the embryo adjacent to the amnionic cavity form the epiblast, whereas cells on the ventral surface adjacent to the yolk sac form the hypoblast.2 By the end of the second week, the embryo thickens cranially to form the prochordal plate, the first morphological feature of craniocaudal orientation.
During the second week, the embryo undergoes gastrulation, which converts the embryo from a two- to a three-layered structure containing ectoderm, mesoderm, and endoderm. During early gastrulation, the primitive streak develops at the caudal end of the embryo and elongates cranially over 3 days (Fig. 1–4). At its full length, the primitive streak occupies the midline in the caudal half of the embryo. Subsequently the primitive streak becomes progressively shorter, regressing back toward the caudal pole of the embryo.2 Throughout gastrulation cells of the epiblast migrate toward the primitive streak and invaginate through the primitive groove running the length of the primitive streak (Fig. 1–4). The first cells to ingress—while the primitive streak is still elongating—give rise to the embryonic endoderm.3–5 Later, as the streak begins to regress toward the caudal pole, endodermal cells are replaced by prospective mesodermal cells that insinuate themselves between the epiblast and the newly formed endoderm. These ingressing mesodermal cells will give rise to the somitic mesoderm.4,6 The remaining epiblast cells spread out to replace the cells that have ingressed through the primitive groove, forming the ectoderm (both neuroectoderm and surface ectoderm).
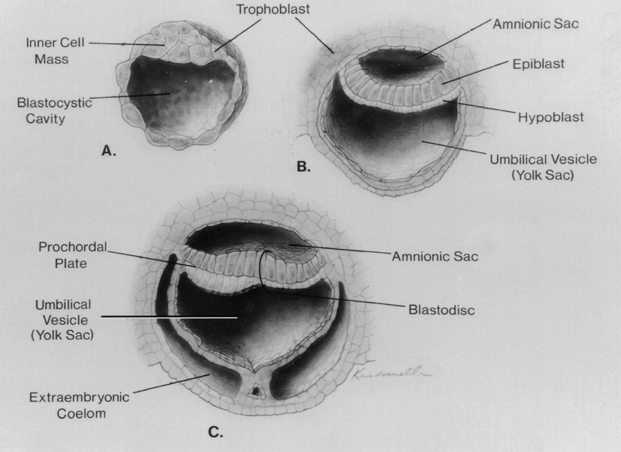
Figure 1–3 Development of the blastocyst; midsagittal illustrations. (A) Continued proliferation of cells produces a sphere containing a blastocystic cavity surrounded by an eccentrically located inner cell mass and a surrounding ring of trophoblast cells. (B) The inner cell mass develops further into a two-layered structure, the blastodisk, containing the epiblast adjacent to the amnionic cavity and the hypoblast adjacent to the yolk sac. (C) With further development, the blastodisk thickens cranially to form the prochordal plate. (Reprinted with permission from Dias MS, Walker ML. The embryogenesis of complex dysraphic malformations: a disorder of gastrulation? Pediatr Neurosurg 1992;18:229–253.)
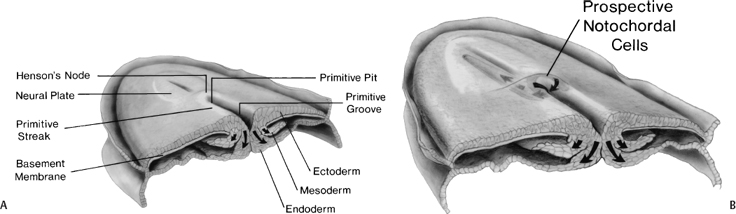
Figure 1–4 Normal human gastrulation. (A) Prospective endodermal and mesodermal cells of the epiblast migrate toward the primitive streak and ingress (arrows) through the primitive groove to become the definitive endoderm and mesoderm. (B) Prospective notochordal cells in the cranial margin of Hensen’s node will ingress through the primitive pit during primitive streak regression to become the notochordal process. (Reprinted with permission from Dias MS, Walker ML. The embryogenesis of complex dysraphic malformations: a disorder of gastrulation? Pediatr Neurosurg 1992;18:229–253.)
The cranial extension of the primitive streak is a specialized structure referred to as Hensen’s node. The caudal extension of the primitive streak is referred to as the primitive pit (Fig. 1–4B). As the primitive streak regresses, mesodermal cells from Hensen’s node ingress through the primitive pit to form the midline notochord (Fig. 1–4B).4,6,7 The notochord continues to elongate as the primitive streak and Hensen’s node regress toward the caudal pole of the embryo, and is flanked bilaterally by the newly developed somitic mesoderm. Together, these embryonic tissues will form the axial skeleton (vertebrae and disks). Both the notochord and the somites are laid down in a rostral to caudal direction. The most caudal vertebrae are formed later from somites derived from the caudal cell mass (the remnants of the primitive streak) and from the caudal notochord derived from the posterior notochordal center located immediately cranial to the caudal cell mass.
Condensation of the Somitic Mesoderm to Form the Somites
Once in its final position the somitic mesoderm aggregates into discrete blocks of tissue, the somites (Fig. 1–5); this process appears to be influenced by, but does not require, the presence of the adjacent notochord and neural tube, because somitic mesoderm, when cultured in isolation, is still capable of generating a discrete, segmented pattern.8,9 In humans, the first somites appear at the beginning of the third embryonic week in the future cervical region.10 Approximately five cranial somites are present by the time the developing neural tube first begins to close; the formation of succeeding caudal somites keeps pace with the rostral to caudal wave of neurulation.
The patterning of the somites is determined by the interaction of various homeobox genes and their gene products. The specification of a vertebra along the craniocaudal axis is thought to be due to its Hox profile—the degree of expression of various homeobox genes at that particular craniocaudal level. Misexpression of one or another homeobox gene in mice can result in either anterior or posterior transformation of various vertebrae. For example, the overexpression of Hoxa-7 results in a posterior translocation of the cervical vertebrae such that the last occipital somites, rather than contributing to the occiput, form an aberrant “proatlas.” The true atlas, which normally would consist of only a ring and whose cells ordinarily would contribute to the formation of the dens, instead expresses a full vertebral body. In contrast, overexpression of the gene Hox-6 results in an anterior spinal translocation, creating a thoracic vertebra that has a rib from the upper lumber vertebra.11 The misexpression of homeobox genes in humans could similarly account for such malformations as the occipitalized atlas, cervical ribs, and lumbarized or sacralized lumbosacral vertebrae.
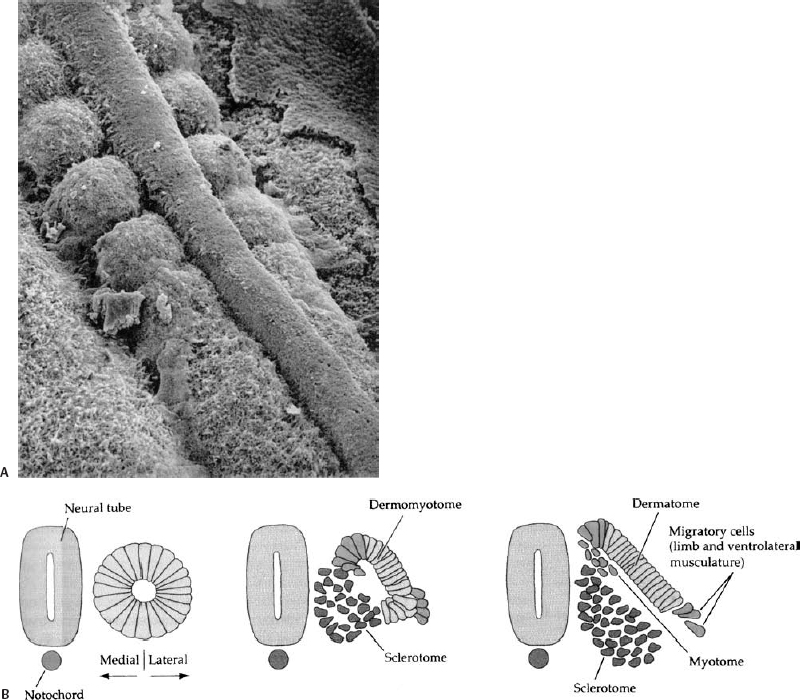
Figure 1–5 Formation of somites. (A) Scanning electron micrograph of chick embryo showing the somitic mesoderm forming as blocks of tissue from the unsegmented somitic mesoderm, lying on either side of the midline neural tube. (B) The somite initially forms immediately lateral to the neural tube and notochord. Reorganization within the somite forms the dorsolateral dermomyotome, and the ventromedial sclerotome that will give rise to the vertebrae.
The maximum number of somites in the human embryo is generally given as 42 to 44, although no more than 38 or 39 are required for the formation of the axial skeleton.10 Most of the “overage” is due to coccygeal somitic segments that disappear during subsequent growth, although a rearrangement or loss of the most cranial segments can occur as well.12 The number and size of the somites appear to be species specific and relatively constant within species.13 If somites are experimentally removed, the embryo is capable of compensating (regulating) to generate a normal number of somites of normal size.14,15
Vertebral malformations of the type seen in clinical practice are rare after experimental excision of somites, suggesting that such malformations may arise later in embryogenesis.
Formation of the Sclerotome and Dermomyotome
The developing somite becomes reorganized dorsoventrally into two segments: a more ventral sclerotome, which will form the axial skeleton, and a more dorsal dermomyotome, which will form the dermis and subcutaneous tissues of the back as well as the myocytes of the dorsal trunk musculature (Fig. 1–5).9 The sclerotome and dermomyotome are readily identified by the expression of various molecular markers. The sclerotome is characterized by the expression of Pax-1 and Pax-9, whereas the dermomyotome is characterized by the expression of Pax-3 and Pax-7 as well as Myo-D and other molecular species.16 The formation of the sclerotome and dermomyotome is regulated by the notochord and/or neural tube floor plate (the ventral portion of the neural tube); a dorsally implanted notochord or floor plate represses the formation of the dermomyotome and instead induces an additional sclerotome, whereas the excision of the notochord inhibits formation of the sclerotome ventrally.9
The subsequent development of the somites can be divided into three phases: the membranous phase during the fifth week of embryogenesis, the chondrification phase beginning at the sixth embryonic week, and the ossification phase beginning at around the ninth embryonic week.
Formation of the Membranous Somite and Resegmentation
The membranous phase begins during the fifth week of embryogenesis. Sclerotomal cells from each somitic pair migrate toward the midline and surround the notochord ventrally and the neural tube dorsally. The merging of cells from somites on either side of the notochord produces the vertebral centra, which first become visible in human embryos during the fifth week. Each sclerotome is divided into cranial and caudal halves, each having a unique histological appearance and expressing a different set of cellular and molecular markers.9 This craniocaudal polarity begins during somite formation and is restricted to the sclerotomal portion of the somite.16 The cranial portion of each sclerotome contains more loosely packed cells, and the caudal portion contains more densely packed cells. Between the cranial and caudal portions lies a hypocellular cleft, called the fissure of von Ebner. The craniocaudal organization of the sclerotome is critical to axonal outgrowth because the outgrowth of spinal nerves at each level of the neuraxis is restricted to the more loosely packed cranial portion of the sclerotome.8,16 The dorsal vertebral arch appears to be exclusively derived from the caudal, more densely packed, half of the sclerotome.
There has been ongoing debate about whether each sclerotome forms a single vertebral centrum or whether the caudal half (the dense-celled portion) of one sclerotome and the cranial half of the adjacent sclerotome combine to form a single vertebral body, with the hypocellular fissure of von Ebner contributing to the intervening intervertebral disk (a process called resegmentation, or Neugliederung). Resegmentation was originally proposed by Remak17 in 1855 to account for the anatomical arrangement of the vertebral centra, dorsal vertebral arch, and spinal nerves. Because at each sclerotomal level the spinal nerve passes through the cranial half-sclerotome and the posterior vertebral arch is derived from the caudal half-sclerotome, one would predict that the spinal nerve would exit cranial to the corresponding pedicle. The observation that each spinal nerve passes caudal to the corresponding pedicle could only be accounted for by resegmentation such that the cranial, loose-celled region of one sclerotome would join with the caudal, dense-celled region of the next more cranial sclerotome to form a single vertebral unit (Fig. 1–6).
The concept of resegmentation has been opposed by Verbout,18 Theiler,19 and others, who have suggested that each vertebra (including the vertebral centrum, posterior arch, transverse processes, ribs, and a single adjacent intervertebral disk) is derived exclusively from a single pair of somites. However, much (but not all) of the experimental evidence supports the concept of resegmentation.20–22 For example, chick–quail chimeras (in which single chick somites are excised and replaced with quail vertebrae) and retroviral-mediated gene transfer paradigms (in which a recombinant retrovirus expressing a -galactosidase marker is injected into single somites) have been used to follow the fate of the labeled somite over time. In these experiments, each somite contributes to two adjacent vertebral centra.
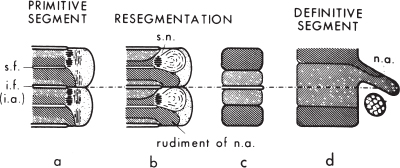
Figure 1–6 Resegmentation of the somites to form the definitive vertebrae. The densely hatched area is the dense-celled area, the more lightly hatched area the loose-celled area. For details, see text. s.f., segmental fissure (of von Ebner); i.f. and i.a., intersegmental fissure and artery; s.n., ; n.a., neural arch. (Reprinted with permission from Tanaka T, Uhthoff HK. Significance of resegmentation in the pathogenesis of vertebral body malformation. Acta Orthop Scand 1981;52:331–338.)
Chondrification Phase
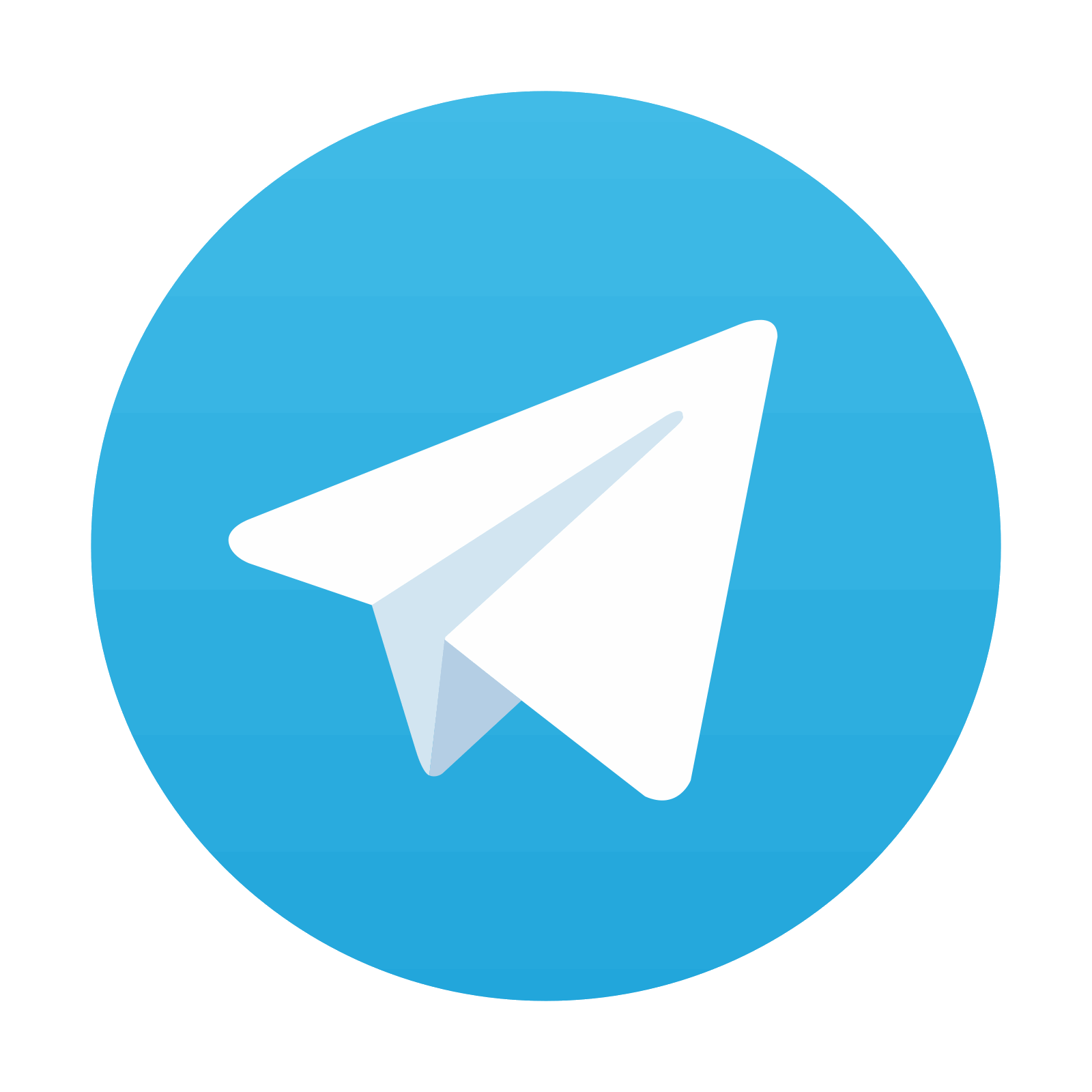
Stay updated, free articles. Join our Telegram channel
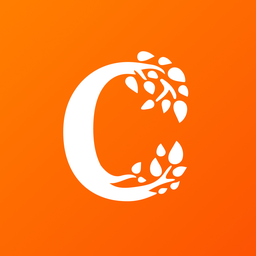
Full access? Get Clinical Tree
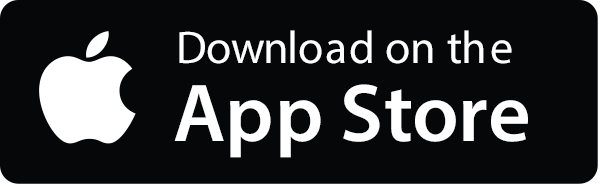
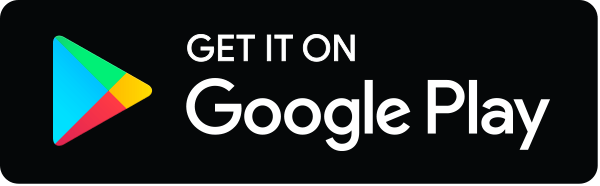