6 Lasers in Facial Plastic Surgery
Introduction
Around the turn of the century, Einstein theorized the processes that must take place for a laser to radiate energy in a publication titled “The Quantum Theory of Radiation.” 1 The first laser was built in 1960 by Maiman. 2 Since then there has been an explosion of laser technology development leading to a great diversity of lasers that encompasses the entire electromagnetic spectrum. Increasingly, lasers are being coupled to other technologies including imaging systems, robotics, and computers to improve delivery of the laser to its target. Through the marriage of the fields of physics and bioengineering, medical lasers have become an important part of the armamentarium of surgeons in the treatment of disease. Initially these lasers were cumbersome and utilized only by surgeons who had been specially trained in the physics of lasers. In the last 20 years medical laser design has evolved toward expanding applications and ease of use, and many surgeons learn the fundamentals of laser physics during residency training or through continuing medical education programs.
In this chapter we discuss laser biophysics, laser–tissue interactions, lasers currently used in facial plastic and reconstructive surgery, general laser safety precautions, and future considerations in the field of cutaneous laser surgery.
Laser Biophysics
Lasers emit light energy that travels in a waveform, as does ordinary light ( Fig. 6.1 ). The wavelength is the distance between two successive peaks of a wave. The amplitude is the height of the peak and is related to the intensity of the light. Frequency (or period) is the amount of time required for one full wave cycle.

To understand how a laser functions it is important to review quantum mechanics. The term laser is an acronym that stands for light amplification by stimulated emission of radiation. If a photon (a unit of light energy) strikes an atom, it will boost one of its electrons to a higher energy level. The atom in this excited state is unstable and will re-emit a photon as the electron drops to its original lower energy level. This is known as spontaneous emission ( Fig. 6.2a ). If an atom—while in a highenergy state—is struck by yet another photon, it will emit two photons that have the same wavelength, direction, and phase when the electrons revert to lower energy levels. One can therefore see how a chain reaction could proceed from successive collisions, generating large volumes of coherent light. This process is known as stimulated emission of radiation and is the underlying principle of laser physics ( Fig. 6.2b ).

Regardless of type, all lasers contain four main components: an excitation mechanism or power source, a lasing medium, an optical cavity or resonator, and a delivery system ( Fig. 6.3 ).

Most clinical lasers used in facial plastic surgery use electrical excitation mechanisms. Some lasers (e.g., flashlamp-excited dye laser) use light as an excitation mechanism. Others may use highenergy radio frequency waves, and still others may use chemical reactions to provide the energy for excitation. The excitation mechanism pumps energy into the resonating chamber that contains a lasing medium that may be solid, liquid, gas, or semiconductive material. At each end of the resonating chamber is a mirror, each facing the other. The energy dumped into the resonating chamber elevates electrons of the lasing medium atoms to a higher energy state. When half the atoms in the resonating chamber have reached this highly excited state, a population inversion has occurred. Spontaneous emission occurs with photons radiating in all directions, with some striking atoms in an already excited state, leading to stimulated emission of paired photons. Amplification of stimulated emission takes place as the photons that travel along the axis between the mirrors are preferentially reflected back and forth between the mirrors. This leads to further stimulation as these photons strike other excited atoms. One mirror is 100% reflective and the other is partially transmissive, allowing some of the radiant energy to escape from the resonating chamber. This laser energy is transmitted to the intended tissues by a delivery system. Most lasers have a flexible fiberoptic delivery system. A notable exception is the carbon dioxide (CO2) laser, which has traditionally been delivered via a rigid articulated arm system. Flexible waveguides for use with the CO2 laser are available but they limit the spot size and power output.
Rather than emanating from the source in all possible directions, the photons in a laser beam are densely packed and traveling in the same direction (collimated). In other words, laser light diverges very little as it travels, giving laser energy its constant intensity even over long distances. The energy is more organized and intense. Because the lasing medium is one kind of molecule or atom, the photons from stimulated emission share the same wavelength, thus creating the quality of monochromaticity. Not only do photons of laser light move in the same direction, they are also in the same temporal and spatial phase. This is called coherence. The properties of monochromaticity, collimation, and coherence differentiate laser light energy from the disorganized and unfocused energy of ordinary light ( Fig. 6.4 ).

Laser–Tissue Interaction
A laser can exert a spectrum of effects on biologic tissue, from modulation of biological function to vaporization ( Fig. 6.5 ). At this time the majority of clinically relevant systems use the laser’s thermal effects to coagulate or vaporize tissue. In the future lasers may be used in a nonthermal capacity as probes to manipulate cellular function without cytotoxic side effects.

The effect of a particular laser on a specific tissue depends on the following three factors: tissue absorption, laser wavelength, and laser energy density. The first two factors are inherent to the tissue and laser, respectively. The third factor, energy density, can be manipulated by the surgeon.
As a laser beam impacts tissue its energy may be absorbed, reflected, transmitted, or scattered ( Fig. 6.6 ). In any particular laser–tissue interaction all four processes occur in varying degrees, with absorption being the most important. The extent of absorption depends on the chromophore content of a tissue. Chromophores are substances that absorb the energy of a particular light wavelength efficiently. For example, CO2 laser energy is absorbed by soft tissues of the body. The target chromophore is water, which makes up 80% of soft tissue content. In contrast, the CO2 laser has a relatively minimal effect on bone (which has a low water content). Initially, as a tissue absorbs laser energy, its molecules begin to vibrate. Absorption of additional energy causes protein denaturation, coagulation, and, finally, vaporization/ablation.

When tissue reflects laser energy, the tissue is unaffected because energy fails to penetrate the surface. Similarly, if laser energy is transmitted through overlying tissues to a deeper layer, the intervening tissue is unaffected. If a laser beam is scattered within tissue, the energy is not absorbed at the surface but is dispersed in deeper layers in a random fashion with dramatically diminished intensity.
The third factor affecting laser–tissue interaction is energy density. Energy density is equal to the power density of the incident beam multiplied by the exposure time. Power density is the power expressed in watts divided by the crosssectional area of the laser beam (spot size), as in the following equation:

Power density may be seen as the amount of power (in watts) delivered to the area irradiated by the laser. Energy density adds the dimension of time:
Energy density = power density × time
Therefore, in a laser–tissue interaction, varying the spot size or the exposure time can alter the tissue effects (if all other factors are held constant). As the spot size of a laser beam decreases (thereby increasing the power density) the amount of power reaching that particular volume of tissue increases. In other words, the photons are packed into a tighter beam width, and their power is dispersed over a smaller volume of tissue. Conversely, as the spot size increases, the energy density of a laser beam decreases. To change the spot size the delivery system can be focused, prefocused, or defocused on the tissue. With both the prefocused and defocused beams, the spot size is larger than the focused beam, leading to less power density.
Another method by which the surgeon can alter tissue effects is pulsing the laser energy. All pulsed modes of irradiation alternate periods of power on with periods of power off ( Fig. 6.7 ). Because energy is not reaching tissue during the off periods, heat is allowed to dissipate during those intervals. If the off periods are longer than the thermal relaxation time of the targeted tissue, there is less risk of damaging the surrounding tissue by the conduction of heat. The thermal relaxation time is the amount of time required for an object to dissipate one half of its heat. The ratio of the on interval to the on-plus-off interval is termed duty cycle.


There are a variety of pulsed modes available. Energy can be pulsed by setting the time that the laser is on (e.g., 0.1 second). The energy can be shuttered, where the continuous wave is blocked during specified intervals by a mechanical shutter. In a superpulse mode the energy is not simply blocked but is stored within the power supply of the laser during the off interval and then released during the on interval. Thus the peak energy of the superpulse mode greatly exceeds that of the continuous or shuttered modes.
In a Q-switched laser the energy is stored during the off time within the lasing medium. This is accomplished by a shutter mechanism in the resonating chamber between the two mirrors. The shutter in the closed position prevents lasing but allows energy to be stored on either side of the shutter. When the shutter is in the open position, the mirrors interact, causing the emission of a highly energized laser beam. The peak energy of a Q-switched laser is extremely high and has a short duty cycle. A mode-locked laser is similar to a Q-switched laser in that a shutter exists between the two mirrors of the resonating chamber. The mode-locked laser opens and closes its shutter in synchrony with the time required for light to reflect between the two mirrors.
Laser Characteristics
Electrically Excited Lasers
CO2 Laser
The CO2 laser is the most commonly used laser in otolaryngologyhead and neck surgery. Its wavelength is 10,600 nm, an invisible wavelength in the far-infrared (far-IR) range of electromagnetic radiation ( Fig. 6.8 ). A companion heliumneon aiming beam, visible to the naked eye, is necessary to allow the surgeon to visualize the intended area of impact. The lasing medium is CO2 gas. As stated above CO2 laser energy is well absorbed by the water molecules in tissue. Its effects are superficial because of high absorption and minimal scatter. Line-of-sight articulated arms are used to deliver the energy, and may be attached to a surgical microscope for enhanced visualization and precision. Flexible delivery systems have become available that circumvent the limitations of the articulated arm on certain anatomical access (the middle ear, for example).

Nd:YAG Laser
The Nd:YAG (neodymium-doped yttrium aluminum garnet) laser’s wavelength is 1,064 nm, in the near-IR spectral region. This is not visible to the human eye and requires the heliumneon aiming beam. Most tissues in the body do not absorb this wavelength well, but pigmented tissue absorbs it better than nonpigmented tissue. It is transmitted through the superficial layers of most tissues and is scattered into the deeper layers.
Compared with the CO2 laser, the scatter of the Nd:YAG is considerably greater. Therefore, the depth of penetration is greater and the Nd:YAG is well suited for coagulating deeper vessels. Experimentally, the depth of maximum coagulation is about 3 mm (at a coagulation temperature of 60°C). 3 , 4 Apfelberg et al 5 report good results in treatment of perioral deep capillary and cavernous lesions with the Nd:YAG laser. Others report successful laser photocoagulation of hemangiomas, lymphangiomas, and arteriovenous malformations. 6 , 7 , 8 The increased depth of penetration and nonselective destruction, however, also predisposes a patient to increased postoperative scarring. Clinically, this is minimized by conservative power settings, a pointillistic approach to the lesion, and avoidance of treatment in areas of thin skin. The use of the Nd:YAG in portwine stains has been virtually replaced by the yellow wavelength lasers. It remains a useful adjuvant laser in cases of nodular portwine stains. 9
In both fibroblast cultures and in normal skin in vivo, the Nd:YAG laser has been shown to suppress collagen production. This suggests an advantage of this laser in the treatment of hypertrophic scars and keloids. 4 Clinically, the recurrence rate in keloid excision is high, however, unless vigorous local steroid treatment is used adjunctively. 10
Contact-Tip Nd:YAG Laser
The use of the Nd:YAG laser in the contact mode drastically alters the physics and absorption properties of the laser. The contact tip consists of a sapphire or quartz tip that attaches to the end of the laser fiber. The contact tip is directly applied to tissue and functions as a thermal scalpel to cut and coagulate simultaneously. Since the surgeon is no longer using the original laser wavelength to cut tissue but rather to heat the tip, its applications more closely resemble those of an electrocautery unit rather than the noncontact mode of the Nd:YAG. Therefore, the principles of laser–tissue interaction do not apply. A wide range of soft tissue surgery using the contact-tip laser has been reported. The response time of the contact-tip laser is not immediate like the free fiber, and therefore there is a lag time for heating up and for cooling down. With experience, however, this laser is useful for raising cutaneous and muscular flaps.
KTP Laser
The KTP (potassium titanyl phosphate) laser is a Nd:YAG laser whose frequency is doubled (wavelength halved) by passing the laser energy through the KTP crystal. This results in a green light (wavelength 532 nm) that corresponds to an absorption peak of hemoglobin. Its tissue penetration and scattering effects are intermediate between the CO2 and the Nd:YAG lasers. The laser energy is delivered by a fiber. In the noncontact mode the laser vaporizes and coagulates. In the semicontact mode the tip of the fiber barely touches the tissue as it becomes a cutting instrument. The higher the energy setting used, the more the laser behaves like a hot knife, analogous to the CO2 laser. Lower energy settings are used primarily to coagulate.
Argon Laser
The argon laser is a visible wavelength laser that has a range from 488 to 514 nm. The makeup of the resonating chamber and the molecular structure of the lasing medium results in a band of wavelengths produced by this type of laser. Filters may then be used to limit the output to a single wavelength. Like the KTP laser, argon laser energy is well absorbed by hemoglobin and its scattering effect is intermediate between the CO2 and the Nd:YAG lasers. Because of the high absorption of hemoglobin, vascular lesions of the skin absorb argon laser energy. The delivery system for the argon laser is a fiberoptic carrier.
Copper Vapor Laser
The copper vapor laser is a visible wavelength laser that produces two separate wavelengths: a pulsed green wavelength at 511 nm and a pulsed yellow light at 578 nm. The laser medium is copper that is excited (vaporized) electrically. A fiberoptic system delivers the energy to a handpiece that has variable spot sizes ranging from 150 to 1,000 µm. The exposure time is variable from 0.075 seconds to continuous operation. The time between pulses is also variable from 0.1 to 0.8 seconds. The yellow light of the copper vapor laser is used in treating benign vascular lesions of the face. The green wavelength may be used to treat pigmented lesions such as freckles, nevi, and keratoses.
Diode Laser
Diodes using superconducting materials have been directly coupled to fiberoptic delivery devices resulting in laser light emission of varying wavelengths (depending on the characteristics of the materials used). The characteristic of diode lasers is their efficiency, as well as the portability of some. Diode lasers can convert incoming electrical power to light at efficiencies of 50%. This efficiency results in less heat production and power input and allows compact diode lasers to be constructed without large cooling systems.
Flashlamp Excited Lasers
Flashlamp-Excited Dye Laser
The flashlamp-excited dye laser (FEDL) was the first medical laser designed specifically to treat benign, vascular cutaneous lesions. It is a visible light laser with a wavelength of 585 nm. This wavelength closely coincides with the third absorption peak of oxyhemoglobin (577 nm), and therefore the laser energy from the FEDL is preferentially absorbed by hemoglobin. In the 577- to 595-nm range there is also less absorption by competing chromophores such as melanin, and less scatter of the laser energy in the dermis and epidermis.
The laser medium is a rhodamine dye that is excited optically by a flashlamp, and the delivery system is a fiberoptic carrier. The handpiece of the FEDL has an interchangeable lens system that allows the use of 3-, 5-, 7- or 10-mm spot size. The pulsewidth of 450 µseconds was chosen based on the thermal relaxation time of ectatic vessels found within benign vascular cutaneous lesions. However, more recent advancements in the area of the pulsed dye lasers have explored lengthening the wavelength to 600 nm for deeper tissue penetration and treatment of resistant portwine stains. This has required the increase of the pulse duration to 1.5 milliseconds in order to achieve the higher fluences required with the longer wavelengths. 11
Although initially designed for the treatment of vascular cutaneous lesions, the FEDL has proven useful in the treatment of scars. Improvement has been noted in scar erythema, texture, and height. 12 In addition, hypertrophic scars treated with this laser have a lower rate of recurrence than those treated with the Er:YAG and CO2 lasers. 13
Erbium Laser
The Erbium:YAG laser takes advantage of the 3,000-nm band of the water absorption spectrum. Its wavelength (2,940 nm) results in strong tissue water absorption (about 12 times that of the CO2 laser). This laser, which is in the near-IR spectrum, is invisible and must be used with a visible aiming beam. The laser is a flashlamp-pumped laser, macropulsed with pulsewidth ranges from 200 to 300 microseconds, which themselves are composed of trains of micropulses. These lasers are used with a handpiece attached to an articulated arm. Scanners can also be incorporated into the system, allowing for more rapid and even removal of tissue.
Ruby Laser
The ruby laser is a flashlamp-pumped laser emitting light at 694 nm. This laser, located in the red portion of the spectrum, is visible. It can be Q-switched to achieve short pulse widths and deep tissue penetration (greater than 1 mm). The long pulse ruby laser is used to deliver preferential heating to hair follicles for hair removal. This laser is transmitted using mirrors and an articulated arm system. It is not well absorbed by water but is strongly absorbed by melanin. Various pigments in tattoos also absorb the 694-nm beam.
Alexandrite Laser
The alexandrite laser, a solid state laser that can be flashlamp pumped, has a wavelength of 755 nm. This wavelength is not visible and thus requires an aiming beam. It is absorbed by blue and black tattoo pigments as well as by melanin but not by hemoglobin. It is a relatively compact laser and can be delivered with a flexible light guide. This laser achieves relatively deep penetration, making it a useful tool in hair and tattoo removal. Seven- and 12-mm spot sizes are available.
Filtered Flashlamp Intense Pulsed Light
Although not a laser, intense pulsed light (IPL) is currently being used to treat a variety of skin conditions including telangiectasias and skin discolorations, as well as for hair removal and for combating the effects of photoaging. It is an intense, noncoherent, pulsed spectrum. The system utilizes crystal filters to emit light with wavelengths of 590 to 1,200 nm. The pulsewidths and fluences, also adjustable, do satisfy the criteria for selective photothermolysis. Based on the patient’s skin type one may customize the wavelengths, number of pulses, duration of pulses, delay between pulses, and power delivered to best match the relative depth, size, and absorption characteristics of the intended target. Customization also minimizes damage to the areas that need to be preserved. This allows for a wide range of patients to benefit from this therapy. IPL intervention has been more useful for prevention of the effects of photoaging and therapy often requires maintenance treatments.
Laser Applications
Vascular Lesions
The vascular lesions of the face and neck differ in cause and natural history. They do, however, share important characteristics that allow them to be selectively treated by lasers. Lasers useful in this area can selectively target the abnormal vessels while sparing surrounding tissue. The skin pigment melanin has an absorption profile similar to that of hemoglobin, thus there is a risk of pigmentary changes in normal tissue around the lesion. The specific lesions discussed include portwine stains, telangiectasias, and hemangiomas.
Port-Wine Stains
Port-wine stains, which are more correctly termed congenital capillary vascular malformations, are benign vascular lesions that commonly involve the face and neck. Approximately 5% of portwine stains are associated with Sturge-Weber syndrome and Klippel-Trenaunay syndrome. 14 Port-wine stains are usually apparent at birth. They usually appear as flat, pink-to-red lesions. As the patient ages they often become darker in color and develop a thickness and nodularity of their surface. Hemangiomas, on the other hand, are not present at birth and often undergo spontaneous involution. Histologically, portwine stains are comprised of large ectatic vessels located in the reticular dermis. Hemangiomas have endothelial hyperplasia in the ectatic vessels. This distinguishes them histologically from portwine stains. 15
Laser Selection
Some surgeons have used the CO2 laser to treat portwine stains. 16 , 17 , 18 However, to eradicate the dermal vessels the overlying epidermis and a portion of the dermis must be likewise vaporized and this can lead to scarring and hypopigmentation. 19 The argon laser has been used extensively for the treatment of portwine stains because its blue-green light in the 488- to 514-nm range is absorbed by oxyhemoglobin. 20 , 21 A variety of treatment techniques using the argon laser have been described. Regardless of technique hypertrophic scarring has been a complication. 22 , 23 , 24 Differing degrees of success in treating portwine stains with the KTP laser 25 and the Nd:YAG laser 7 have been reported. In a study of 107 patients of Chinese descent treated with PDL or Nd:YAG, Ho et al reported that these patients required an average of 6.1 treatments for maximal lesional resolution. 26 Another study of 22 Chinese patients found that the Nd:YAG laser was only partially effective and required higher fluences for optimal results. 27 The yellow wavelength lasers (FEDL, copper vapor laser) have provided a breakthrough in the treatment of portwine stains. Additionally the nonlaser IPL systems were found to be a highly effective and safe treatment for PWS by one study. 28 These lasers function based on the principle of selective photothermolysis described by Anderson and Parrish. 29 , 30 This process is the selective heating of the ectatic vessel within a cutaneous lesion by preferential light absorption and heat production. To achieve ablation of a portwine stain, the ectatic vessel must be heated to a temperature high enough to damage the endothelial lining. The yellow wavelength lasers accomplish this by heating the blood within the vessel; the heat is conducted to the vessel wall. Simply coagulating the blood within the vessel without damaging the vessel wall allows recannulation to occur. Localizing the heat to the target vessel and sparing the surrounding tissue is attained by using the proper wavelength and by taking into account the thermal relaxation time of the tissues being treated. The wavelength of the yellow lasers is in the 577-to 585-nm range. The 577-nm wavelength precisely matches the third absorption peak of oxyhemoglobin. In comparison with other laser wavelengths such as the KTP or argon, there is a decrease in absorption by the competing chromophore melanin and less scatter in the dermis and epidermis 31 ( Fig. 6.9 ). Tan et al showed that the FEDL at 585 nm causes deeper penetration of laser energy with no loss of vascular selectivity. 32 With deeper penetration, there is faster clearing of the lesion.

The yellow wavelength lasers use different mechanisms to obtain adequate thermal relaxation times for the ectatic vessels within portwine stains. The FEDL has a set pulse width of 450 µsec; this was calculated on the average diameter of vessels within portwine stains. The copper vapor laser allows for variability in setting the pulse width and the time between pulses. The continuous wave yellow dye laser may be used with a Hexascanner, a device that mechanically varies the position of laser pulses placed within a hexagonal pattern. By placing pulses in nonadjacent areas, the previously treated tissue has time to cool. 33 In theory, longer wavelengths would minimize the number of treatments needed for complete resolution of vascular lesions as dermal scattering decreases allowing for greater penetration. A new generation of pulsed dye lasers with wavelengths between 585 nm and 600 nm has been introduced and shown to be clinically effective in the treatment of portwine stains and telangiectasia. 34 , 35 However, the absorption of oxyhemoglobin decreases significantly at wavelengths longer than 585 nm, requiring an increase in fluence. Increasing the energy targeted at the lesion increases thermal damage to the surrounding normal skin. Thus skin cooling techniques such as cryogen spray cooling were developed. Cooling allows skin to be treated with more than twice the laser energy used in clinical settings without inducing thermal injury to the epidermis. 36 , 37 , 38 Furthermore, the effect of the cryogen cooling spray on the laser–tissue interaction was shown to be negligible. 39 Multiple studies suggest that the optimal results for portwine stains are achieved using the PDL with cryogen cooling. 40 , 41 , 42 Other methods of cooling, such as using a sapphire thermal surface conductor, have shown beneficial in decreasing the transient temperature and related posttreatment purpura seen with the FEDL. 43
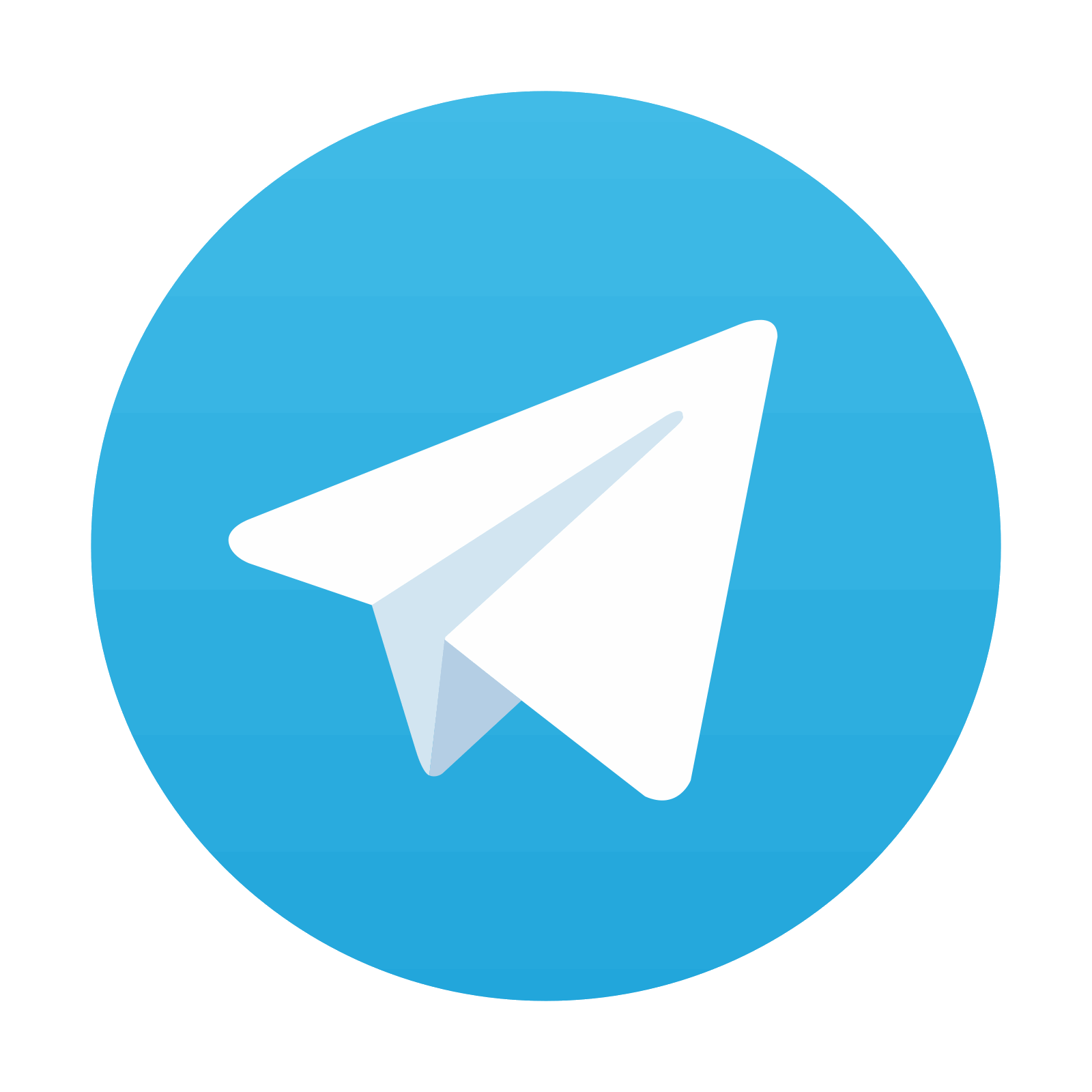
Stay updated, free articles. Join our Telegram channel
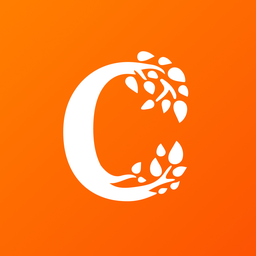
Full access? Get Clinical Tree
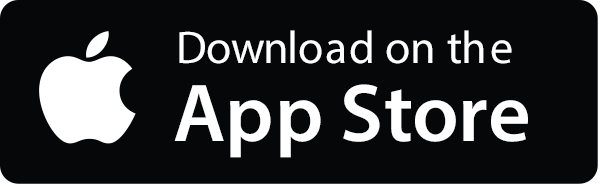
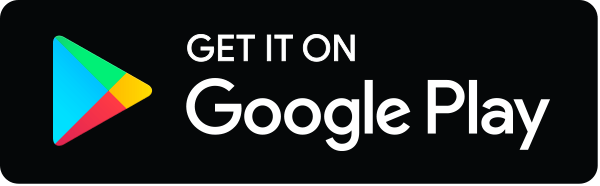
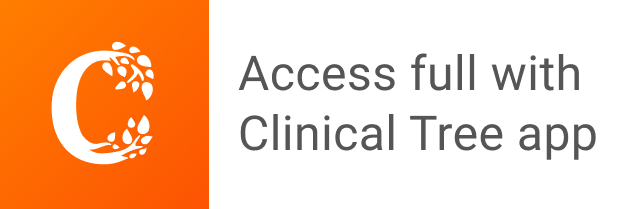