Virus-Mediated Transfer
Several viruses have been used as templates to design gene therapy vectors. Their common feature is their natural ability to infect eukaryotic cells and to carry their nucleic acids directly to the nucleus. They are used to transfer cDNAs, genes or small antisense sequences. These viruses are not equivalent, and Table 140.1 summarizes their main differences relevant for gene therapy.
Table 140.1 Gene therapy viral vectors
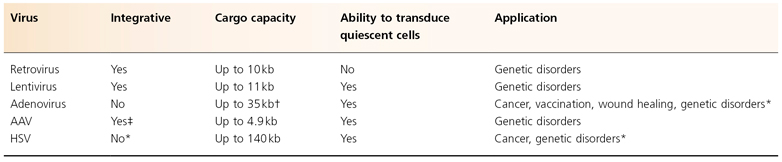
* The use of phage integrases or transposases such as ϕC31 or sleeping beauty could confer on these vectors the ability to integrate the therapeutic gene into the target cell’s genome.
† The development of ‘gutless’ adenoviral vectors has improved the cargo capacity from 9 to 35 kb.
‡ AAV vectors lack the rep protein and thus integration is less efficient than with the wild-type virus.
Retroviruses
Retroviruses belong to the Retroviridae family, which also comprises the lentiviruses such as human immunodeficiency virus (HIV). Retroviral vectors, the most widely used vectors for gene therapy, are derived from the Moloney murine leukaemia virus (MoMuLV). To generate replication-defective MoMuLV, all the protein-encoding sequences are removed from the virus and replaced by the transgene of interest. However, the sequences required for encapsidation of the vector RNA, the ψ packaging signal, have to be included in the vector construct.
Retroviruses are able to transduce a large range of cell types with a high efficiency and for a long time. Progress in the design of retroviral vectors has permitted the transfer of cDNA as large as 10 kb, and the vectors can be pseudo-typed to enlarge the spectrum of potential target cells. This means that different envelope proteins can be used, each of which has its own advantages and disadvantages. For example, the glycosylated protein of the vesiculous stomatitis virus (VSV-G) is commonly used because it allows the targeting of a wide spectrum of cells. VSV-G mediates viral entry by membrane fusion via the interaction with phospholipid components of the cell membrane, and therefore it has a broad host range. Moreover, it is made of one monomer only and thus is more resistant than the MoMuLV natural amphotropic envelope protein. This resistance offers the possibility of ultracentrifuging the viral supernatant to obtain higher titres, about 100–300-fold concentrated.
The major disadvantage of using VSV-G as an envelope is its toxic effects in the packaging cells, and in some cases the use of the amphotropic protein is required. Retrovirus integrate the genome only in dividing cells. Thus, one of the limitations of these vectors is that quiescent cells cannot be targeted. The other major issue is that the transgene is randomly integrated into the cell genome. This can lead to serious adverse effects such as the occurrence of leukaemia associated with the X-severe combined immunodeficiency (SCID) gene therapy trial [1,2]. The development of self-inactivating (SIN) vectors, in which the enhancer located in the U3 region of the 3′-long-term repeat (LTR) has been deleted, offers a greater biosafety and should prevent oncogenic events due to an upregulation of a proto-oncogene in the close vicinity of the integration site. Recent data clearly demonstrate that transcriptionally active (i.e. non-SIN) LTRs are the major determinants of genotoxicity in retroviral and lentiviral vectors, and that SIN vectors have greater therapeutic potential [3–5]. Moreover, they allow for the use of tissue-specific promoters to drive transgene expression.
The drawback of the inactivation of the transcriptional regulatory elements of the proviral LTRs is a substantial loss of viral titre, which was at least 10–100-fold lower than the parental retroviral vector [6,7]. SIN vectors are now mandatory for clinical applications in European countries and in the United States [8].
Lentiviruses
Lentiviral vectors are derived from the human immunodeficiency virus 1 (HIV1). They share almost all their features with the retroviral vectors, with the major exception that they are able to transduce quiescent cells. Like retroviral vectors, their integration is not site specific and thus can theoretically lead to oncogenesis through insertional mutagenesis. As for the retroviral vectors, lentiviral vectors can be pseudo-typed to enlarge the spectrum of target cells. The use of the VSV-G protein also permits the production of higher-titre vector preparations. The development of lentiviral SIN vectors [9,10], like the one developed first for retroviral vectors, greatly reduces this risk [3–5]. SIN lentiviral vectors have been successfully used in several preclinical studies to transfer genes of interest into primary keratinocytes [11–15].
Adenoviruses
Human adenoviruses (Ad) are a group of double-stranded DNA viruses that infect a variety of vertebrate hosts, including rodents, chicken and non-human primates. These are divided into six subgroups according to rather arbitrary criteria of ability to transform rodent cells, pattern of haemagglutination, and GC contents of the DNA. In the context of gene therapy, serotypes 5 and 2 of the subgroup C have been used almost exclusively because these are the serotypes about which most is known regarding structure and biology, and convenient biological reagents are available to produce recombinant subgroup C Ad gene transfer vectors in large quantities. First, Ad vectors permit limited Ad gene expression and DNA replication, which probably contributes to the immune response against the vector. In addition, the possibility of making replication-competent adenovirus (RCA) during propagation is a potentially dangerous feature. By making additional mutations or deletions in the Ad genome, both of these problems can be avoided. Such second and third generations of Ad vectors avoid the presence of RCA in the preparation. The third-generation Ad vectors have been termed, because of their characteristics, high-capacity, helper-dependent and ‘gutless’ adenoviral vectors. They were designed to minimize the number of Ad viral genes present in the vector, increasing the cloning capacity up to 35 kb and reducing the host immune response against the Ad vector-transduced cells.
Adenoviruses are not oncogenic in humans; in addition, their genomes are completely defined and can easily be modified. Recombinant Ad can readily be produced in large quantities and highly concentrated without modifying the ability of the virus to infect cells. The ability to generate high-titre (1014 particles/mL) recombinant Ad vectors and their efficient transduction of both dividing and non-dividing cells initially boded well for a very successful vector. Unfortunately, cells transduced by recombinant Ad vectors are eliminated by cytotoxic T-lymphocytes (CTLs) generated against viral proteins or the transgene [16,17]. Furthermore, Ad vectors elicit a potent humoral response that effectively eliminates all subsequent transduction. This is even more pertinent to adenoviral vectors because they do not integrate, and hence they suffer loss by cell division and by DNA degradation. The high-titre recombinant Ad vectors are potentially toxic, although the precise mechanism associated with this toxicity remains uncertain. The death of a patient as the direct result of recombinant adenoviral vector therapy is a tragic warning sign that signals the need for further improvement of viral gene therapy. Ad vectors will, however, continue to be used in situations in which a high-level but transient expression of the foreign gene is required.
Adeno-Associated Virus
The human parvovirus adeno-associated virus (AAV) belongs to the Parvoviridae family. It is a dependovirus that needs a helper virus to replicate. Thus, the AAVs are naturally defective for replication. AAV has aroused considerable interest as a potential vector for human gene therapy. Among the favourable properties of the virus are its lack of association with any human disease, the wide range of cell lines derived from different tissues that can be infected and the ability of the virus to integrate into the genome of the infected cell to establish a latent infection. The last property appears to be unique among mammalian viruses because the integration can occur in quiescent cells, albeit at a lesser frequency than in dividing cells, and because AAV integration occurs at a specific site in the human genome. This site, called AAVS1, is located on chromosome 19 (19q13.3–qter). The site specificity of AAV integration is mediated by the AAV rep78 protein or by its C-terminally spliced variant Rep68 [18,19]. Although the inverse terminal repeats (ITRs) are the only genomic elements necessary for integration, efficient integration and site specificity require the presence of the viral Rep protein [20]. Because AAV vectors lack the Rep expression cassette, AAV vectors integrate with low efficacy and low specificity into the host genome. Integration of recombinant AAV (rAAV) has been observed in dividing cells [21], as well in non-dividing cells in vitro [22].
Apart from integration into the host genome, the presence of episomal forms of rAAV has been demonstrated in vivo [23–25]. Several studies have demonstrated the presence of AAV vectors DNA in an episomal circular form in muscle and brain tissue transduced with AAV vectors [23–25]. Because this form of episomal AAV DNA persists for months and maybe years, episomal AAV DNA might be a major contributor to the long-term expression of transgenes delivered by AAV vectors. The major disadvantage of the AAV vector is its low cargo capacity, between 4.1 and 4.9 kb, but a recent strategy based on hybrid dual AAV vector has allowed the transfer of the 6 kb minidystrophin cDNA [26]. Nonetheless, AAV are very valuable vectors which are now extensively used to transfer small antisense sequences to selectively knock down alleles or modulate the splicing of target genes [27,28].
Single administration of rAAV into the muscle of immunocompetent mice does not result in a detectable cellular immune response; however, a humoral response can be detected. Pre-existing humoral immunity against AAV is likely to be a hurdle for many gene therapy approaches in humans. Thus, and especially if vectors are readministered, efforts to overcome humoral immunity against AAV have to be considered.
Herpes Simplex Virus Amplicons
Transfer of large DNA constructs in gene therapy studies is being recognized for its importance in maintaining the natural genomic environment of the gene of interest and providing tissue-specific regulation and control. Recent advances in the manipulation of BAC (bacterial artificial chromosomes) and PAC (P1-derived artificial chromosomes) inserts by homologous recombination in bacteria have increased their versatility as cloning systems for genomic DNA. However, the use of these vectors in gene expression studies is hampered by the difficulty of transferring and retaining intact sequences of genomic DNA over 100 kb long in human cells. Although gene expression from the genomic DNA inserts of BACs and PACs has been demonstrated in cell culture systems and in transgenic animal models, the efficiency of physical methods of intact DNA delivery is usually low for DNA constructs more than 100 kb long [29]. Mecklenbeck et al. successfully corrected the type VII collagen expression in immortalized keratinocytes from an recessive dystrophic epidermolysis bullosa (RDEB) patient using a microinjected PAC containing the entire COL7A1 locus (32 kb), demonstrating the ‘proof of principle’ of genomic DNA vectors as a means of restoring expression of gene as large as COL7A1 [30].
Viral vectors are an efficient means of delivering genes to cells, but the size of most genomic loci generally excludes their use in the context of viral vectors. In contrast, the large size (152 kb) of herpes simplex virus type 1 (HSV-1) confers a transgene capacity that can accommodate many genomic loci. The capacity of HSV-1 exceeds that of the helper-dependent or ‘gutless’ adenoviral vectors, which have been used to express a genomic DNA locus of 19 kb in size. Infectious amplicon plasmids carrying an origin of replication (oris) and the packaging/cleavage signal (pac) from HSV-1 have been widely used for gene delivery both in vitro and in vivo [31,32]. Furthermore, the manipulation of HSV-1 in bacteria has led to the development of helper virus-free packaging systems for HSV-1 amplicons capable of high-titre amplicon production [33]. HSV-1 amplicons do not express any viral genes but depend on helper functions for replication and packaging into virions. The problems of cytotoxicity and antigenicity are largely reduced when the helper functions are provided by a packaging-defective HSV-1 genome, which results in vector stocks that are essentially free of contaminating helper virus. However, the problem remains that it is difficult to achieve stable transgene expression with HSV-1-based vectors, as with other viral vectors.
The human herpes viruses represent promising candidate vectors for several types of gene therapy applications, which include neuropathological disorders, cancer, pain control, autoimmune syndromes and metabolic diseases. The primary tropism of HSV is the neurones, however experimental HSV infection is not limited to neurones. The virus is capable of infecting most mammalian cell types and does not require cell division for infection and gene expression. Accordingly, HSV may be generally useful for gene transfer to a variety of non-neuronal tissues, particularly if short-term transgene expression is required to achieve a therapeutic effect.
The large cargo capacity of the herpes viral vectors permits the transduction of complete loci, preserving the normal gene regulation.
Non-Viral Delivery
Although often effective, viral vectors have significant drawbacks, including concerns about safety, immunogenicity, scalability of production and cost-effectiveness. Non-viral transfer to skin has several key features. First, non-viral therapeutic agents are usually plasmids or oligonucleotides, which are generally inexpensive and easy to construct and manufacture. Second, they have been shown to be effective for certain applications such as immunization, in part because of dendritic and Langerhans antigen-presenting cells located in the skin [34,35]. Third, this approach results in transient and variable levels of gene expression owing to rapid turnover in renewable epithelial tissues [36–38]. In addition, delivery of naked DNA offers a safer way to transfer a therapeutic gene both in vivo or ex vivo, without the risk of inducing unwanted side-effects including infection, mutagenesis or generation of immune response against viral proteins, which can limit subsequent readministrations. The principal issues of non-viral gene therapy are the low efficiency of gene transfer, the inability to selectively deliver DNA to specific cell types and the variable levels of gene expression.
Tremendous progresses have been made in the past 5 years in the development of efficient non-viral delivery methods. This is in part due to the rise of new RNA-based therapeutic approaches involving small antisense sequences such as RNA interference (RNAi), modulation of pre-mRNA splicing or trans-splicing (for review see [39]). In vivo delivery systems for siRNA include liposomes [40], lipid/alcohol-based formulation cream [41] and nanoparticles [42,43]. Until recently, non-viral gene transfer was not applicable for long-term therapy, as such a strategy would require targeting of stem cells and integration into their genome, which was a very rare event. However, Ortiz-Urda et al. [44] have recently used a polybrene shock to successfully transfer the COL7A1 cDNA into RDEB keratinocytes using the phage ϕC31 integrase, This ex vivo non-viral gene therapy approach combines the advantage of a non-viral system and genomic integration which allows for long-term expression. However, a recent study showed that ϕC31 expression led to DNA damage and chromosomal aberrations in primary human fibroblasts. These chromosomal rearrangements (translocations, aneuploidy, ring chromosomes) were not due to the puromycin treatment, as cells transfected with the pBabepuro plasmid backbone and selected with this antibiotic did not show abnormal karyotypes [45]. This observation may temper the interest of this approach. Transposable elements such as sleeping beauty have also been used to mediate permanent genetic modification into keratinocytes [46]. Despite their advantages over viral vectors (near-random integration profile), they were not as efficient and antibiotic selection was required to enrich the genetically modified population. The use of engineered hyperactive sleeping beauty transposase, allowing ∼30% of transposition in HeLa cells, may circumvent the need for selection [47]. This has been confirmed recently on more clinically relevant primary cells (CD34+ haematopoietic stem cells and in vivo liver injection), allowing for 35–50% of transposition efficiency [48].
The different non-viral gene delivery methods that have been used for skin gene therapy are illustrated in the In vivo gene therapy section, below.
Approaches to Cutaneous Gene Delivery
Two distinct approaches have been used to genetically modify keratinocytes or fibroblasts. The in vivo approach consists of the direct administration of the genetic material into the skin using either viral or non-viral delivery vectors, whereas in the ex vivo approach, the genetic correction of cells is achieved while they are cultured (see Fig. 140.1).
Ex vivo Gene Therapy
The cells are harvested from the host from a skin biopsy and grown in vitro and the gene is then transferred to the cells while they are growing in tissue culture. After genetic correction, the cells are expanded and grafted back onto the recipient. This approach has many advantages: targeting of the cells of interest, possible selection of the transduced cells and a high efficacy of gene transfer. The disadvantages include the need for a good cell culture method that preserves stem cells for long-term correction, the loss of the skin appendages on the treated area and the fact that the technique is labour intensive, in that it involves extensive tissue culture and grafting efforts. Gene transfer preclinical studies using this approach have been most successfully achieved with integrating retroviral or lentiviral vectors[12,49,50–61]. Non-viral vectors have also been used for ex vivo gene therapy to correct haemophilia A by injection of transfected autologous fibroblasts in a phase I trial [62]. This approach was also used to correct type VII collagen-deficient keratinocytes and laminin 5 deficiency in preclinical studies [44,46]. The grafting efforts have benefited from experience acquired primarily in the treatment of burns and cutaneous ulcers [63–65]. These include refinement of approaches, ranging from the application of simple sheets of epithelium grown in vitro to the use of complex skin-equivalent grafts composed of living cells seeded in natural or synthetic matrices.
In vivo Gene Therapy
This direct approach includes the topical application or the injection of the gene therapy compound into the skin.
Injection
In this approach, the therapeutic agent is directly injected into the skin by several means: intradermal injection of naked plasmid DNA in phosphate-buffered saline (PBS) [37,66], ballistic particle (DNA-coated gold particles) bombardment using a ‘gene gun’ method, also called ‘biolistic delivery’ [67,68], electroporation [69,70], jet injection [71], puncture-mediated transfer [72,73] or tape stripping [74]. Generally, these methods are at present inefficient for gene therapy purposes, but some may be useful for DNA vaccines or anticancer applications. However, in the case of small molecules (siRNA, antisense oligoribonucleotides), the intradermal route of administration has shown some interest for the treatment of genetic skin disorders [75].
Topical Application
The topical route of administration allows for delivery to large areas of the epidermis. The therapeutic compound is applied to the skin, either in a liposome mixture, as uncoated DNA for epicutaneous transfer into the epidermis or complexed with newly identified cell-penetrating peptides [41,76–82]. However, the stratum corneum, which is the outer protective layer of the epidermis, is hydrophobic and acts as a very efficient barrier to large negatively charged molecules such as DNA, even when complexed to liposomes. Other topical methods involve the use of viral vectors such as adenovirus and modified herpes virus [83,84], but this raised safety concerns about viral vector distribution in vivo and immune response towards the vector.
Direct in vivo gene transfer is, in general, less labour intensive than ex vivo approaches and can produce biologically active levels of gene expression and protein production. However, direct approaches have been plagued by low levels of gene transfer, which achieves transgene expression in only a minority of cells for periods as short as few days [37,83].
The development of new gene therapy approaches involving small antisense compounds such as siRNA or exon skipping (see below) has raised a new interest in in vivo non-viral topical delivery. These small compounds are easier to deliver through the skin barrier than large plasmids and recent developments in lipid cream formulation, cell-penetrating peptides and nanoparticles are very promising tools for clinical application [41,79,81].
References
1 Hacein-Bey-Abina S, von Kalle C, Schmidt M et al. A serious adverse event after successful gene therapy for X-linked severe combined immunodeficiency. N Engl J Med 2003;348(3):255–6.
2 Hacein-Bey-Abina S, von Kalle C, Schmidt M et al. LMO2-associated clonal T cell proliferation in two patients after gene therapy for SCID-X1. Science 2003;302(5644):415–19.
3 Cornils K, Lange C, Schambach A et al. Stem cell marking with promotor-deprived self-inactivating retroviral vectors does not lead to induced clonal imbalance. Mol Ther 2009;17(1):131–43.
4 Maruggi G, Porcellini S, Facchini G et al. Transcriptional enhancers induce insertional gene deregulation independently from the vector type and design. Mol Ther 2009;17(5):851–6.
5 Montini E, Cesana D, Schmidt M et al. The genotoxic potential of retroviral vectors is strongly modulated by vector design and integration site selection in a mouse model of HSC gene therapy. J Clin Invest 2009;119(4):964–75.
6 Yee JK, Moores JC, Jolly DJ, Wolff JA, Respess JG, Friedmann T. Gene expression from transcriptionally disabled retroviral vectors. Proc Natl Acad Sci USA 1987;84(15):5197–201.
7 Yu JY, DeRuiter SL, Turner DL. RNA interference by expression of short-interfering RNAs and hairpin RNAs in mammalian cells. Proc Natl Acad Sci USA 2002;99(9):6047–52.
8 De Luca M, Pellegrini G, Mavilio F. Gene therapy of inherited skin adhesion disorders: a critical overview. Br J Dermatol 2009;161(1):19–24.
9 Miyoshi H, Blomer U, Takahashi M, Gage FH, Verma IM. Development of a self-inactivating lentivirus vector. J Virol 1998;72(10):8150–7.
10 Zufferey R, Dull T, Mandel RJ et al. Self-inactivating lentivirus vector for safe and efficient in vivo gene delivery. J Virol 1998;72(12):9873–80.
11 Baek SC, Lin Q, Robbins PB, Fan H, Khavari PA. Sustainable systemic delivery via a single injection of lentivirus into human skin tissue. Hum Gene Ther 2001;12(12):1551–8.
12 Chen M, Kasahara N, Keene DR et al. Restoration of type VII collagen expression and function in dystrophic epidermolysis bullosa. Nat Genet 2002;32(4):670–5.
13 Di Nunzio F, Maruggi G, Ferrari S et al. Correction of laminin-5 deficiency in human epidermal stem cells by transcriptionally targeted lentiviral vectors. Mol Ther 2008;16(12):1977–85.
14 Kuhn U, Terunuma A, Pfutzner W, Foster RA, Vogel JC. In vivo assessment of gene delivery to keratinocytes by lentiviral vectors. J Virol 2002;76(3):1496–504.
15 Richard E, Mendez M, Mazurier F et al. Gene therapy of a mouse model of protoporphyria with a self-inactivating erythroid-specific lentiviral vector without preselection. Mol Ther 2001;4(4):331–8.
16 Kafri T, Morgan D, Krahl T, Sarvetnick N, Sherman L, Verma I. Cellular immune response to adenoviral vector infected cells does not require de novo viral gene expression: implications for gene therapy. Proc Natl Acad Sci USA 1998;95(19):11377–82.
17 Tripathy SK, Black HB, Goldwasser E, Leiden JM. Immune responses to transgene-encoded proteins limit the stability of gene expression after injection of replication-defective adenovirus vectors. Nat Med 1996;2(5):545–50.
18 Linden RM, Ward P, Giraud C, Winocour E, Berns KI. Site-specific integration by adeno-associated virus. Proc Natl Acad Sci USA 1996;93(21):11288–94.
19 Linden RM, Winocour E, Berns KI. The recombination signals for adeno-associated virus site-specific integration. Proc Natl Acad Sci USA 1996;93(15):7966–72.
20 Weitzman MD, Kyostio SR, Kotin RM, Owens RA. Adeno-associated virus (AAV) Rep proteins mediate complex formation between AAV DNA and its integration site in human DNA. Proc Natl Acad Sci USA 1994;91(13):5808–12.
21 Yang CC, Xiao X, Zhu X et al. Cellular recombination pathways and viral terminal repeat hairpin structures are sufficient for adeno-associated virus integration in vivo and in vitro. J Virol 1997;71(12):9231–47.
22 Wu P, Phillips MI, Bui J, Terwilliger EF. Adeno-associated virus vector-mediated transgene integration into neurons and other nondividing cell targets. J Virol 1998;72(7):5919–26.
23 Duan D, Sharma P, Yang J et al. Circular intermediates of recombinant adeno-associated virus have defined structural characteristics responsible for long-term episomal persistence in muscle tissue. J Virol 1998;72(11):8568–77.
24 Fisher KJ, Jooss K, Alston J et al. Recombinant adeno-associated virus for muscle directed gene therapy. Nat Med 1997;3(3):306–12.
25 Nakai H, Iwaki Y, Kay MA, Couto LB. Isolation of recombinant adeno-associated virus vector-cellular DNA junctions from mouse liver. J Virol 1999;73(7):5438–47.
26 Ghosh A, Yue Y, Lai Y, Duan D. A hybrid vector system expands adeno-associated viral vector packaging capacity in a transgene-independent manner. Mol Ther 2008;16(1):124–30.
27 Goyenvalle A, Vulin A, Fougerousse F et al. Rescue of dystrophic muscle through U7 snRNA-mediated exon skipping. Science 2004;306(5702):1796–9.
28 Xia H, Mao Q, Eliason SL et al. RNAi suppresses polyglutamine-induced neurodegeneration in a model of spinocerebellar ataxia. Nat Med 2004;10(8):816–20.
29 Compton SH, Mecklenbeck S, Mejia JE et al. Stable integration of large (>100 kb) PAC constructs in HaCaT keratinocytes using an integrin-targeting peptide delivery system. Gene Ther 2000;7(18):1600–5.
30 Mecklenbeck S, Compton SH, Mejia JE et al. A microinjected COL7A1-PAC vector restores synthesis of intact procollagen VII in a dystrophic epidermolysis bullosa keratinocyte cell line. Hum Gene Ther 2002;13(13):1655–62.
31 Geller AI, Breakefield XO. A defective HSV-1 vector expresses Escherichia coli beta-galactosidase in cultured peripheral neurons. Science 1988;241(4873):1667–9.
32 Spaete RR, Frenkel N. The herpes simplex virus amplicon: a new eucaryotic defective-virus cloning-amplifying vector. Cell 1982;30(1):295–304.
33 Saeki Y, Fraefel C, Ichikawa T, Breakefield XO, Chiocca EA. Improved helper virus-free packaging system for HSV amplicon vectors using an ICP27-deleted, oversized HSV-1 DNA in a bacterial artificial chromosome. Mol Ther 2001;3(4):591–601.
34 Falo LD Jr. Targeting the skin for genetic immunization. Proc Assoc Am Phys 1999;111(3):211–19.
35 Raz E, Carson DA, Parker SE et al. Intradermal gene immunization: the possible role of DNA uptake in the induction of cellular immunity to viruses. Proc Natl Acad Sci USA 1994;91(20):9519–23.
36 Choate KA, Khavari PA. Sustainability of keratinocyte gene transfer and cell survival in vivo. Hum Gene Ther 1997;8(8):895–901.
37 Hengge UR, Chan EF, Foster RA, Walker PS, Vogel JC. Cytokine gene expression in epidermis with biological effects following injection of naked DNA. Nat Genet 1995;10(2):161–6.
38 Vogel JC. A direct in vivo approach for skin gene therapy. Proc Assoc Am Phys 1999;111(3):190–7.
39 Wood M, Yin H, McClorey G. Modulating the expression of disease genes with RNA-based therapy. PLoS Genet 2007;3(6):e109.
40 Morrissey DV, Lockridge JA, Shaw L et al. Potent and persistent in vivo anti-HBV activity of chemically modified siRNAs. Nat Biotechnol 2005;23(8):1002–7.
41 Takanashi M, Oikawa K, Sudo K et al. Therapeutic silencing of an endogenous gene by siRNA cream in an arthritis model mouse. Gene Ther 2009;16(8):982–9.
42 Li SD, Chen YC, Hackett MJ, Huang L. Tumor-targeted delivery of siRNA by self-assembled nanoparticles. Mol Ther 2008;16(1):163–9.
43 Patel PC, Giljohann DA, Seferos DS, Mirkin CA. Peptide antisense nanoparticles. Proc Natl Acad Sci USA 2008;105(45):17222–6.
44 Ortiz-Urda S, Thyagarajan B, Keene DR et al. Stable nonviral genetic correction of inherited human skin disease. Nat Med 2002;8(10):1166–70.
45 Liu J, Skjorringe T, Gjetting T, Jensen TG. PhiC31 integrase induces a DNA damage response and chromosomal rearrangements in human adult fibroblasts. BMC Biotechnol 2009;9:31.
46 Ortiz-Urda S, Lin Q, Yant SR, Keene D, Kay MA, Khavari PA. Sustainable correction of junctional epidermolysis bullosa via transposon-mediated nonviral gene transfer. Gene Ther 2003;10(13):1099–104.
47 Baus J, Liu L, Heggestad AD, Sanz S, Fletcher BS. Hyperactive transposase mutants of the Sleeping Beauty transposon. Mol Ther 2005;12(6):1148–56.
48 Mates L, Chuah MK, Belay E et al. Molecular evolution of a novel hyperactive Sleeping Beauty transposase enables robust stable gene transfer in vertebrates. Nat Genet 2009;41(6):753–61.
49 Choate KA, Kinsella TM, Williams ML, Nolan GP, Khavari PA. Transglutaminase 1 delivery to lamellar ichthyosis keratinocytes. Hum Gene Ther 1996;7(18):2247–53.
50 Choate KA, Medalie DA, Morgan JR, Khavari PA. Corrective gene transfer in the human skin disorder lamellar ichthyosis. Nat Med 1996;2(11):1263–7.
51 Dellambra E, Pellegrini G, Guerra L et al. Toward epidermal stem cell-mediated ex vivo gene therapy of junctional epidermolysis bullosa. Hum Gene Ther 2000;11(16):2283–7.
52 Dellambra E, Prislei S, Salvati AL et al. Gene correction of integrin beta4-dependent pyloric atresia-junctional epidermolysis bullosa keratinocytes establishes a role for beta4 tyrosines 1422 and 1440 in hemidesmosome assembly. J Biol Chem 2001;276(44):41336–42.
53 Dellambra E, Vailly J, Pellegrini G et al. Corrective transduction of human epidermal stem cells in laminin-5-dependent junctional epidermolysis bullosa. Hum Gene Ther 1998;9(9):1359–70.
54 Freiberg RA, Choate KA, Deng H, Alperin ES, Shapiro LJ, Khavari PA. A model of corrective gene transfer in X-linked ichthyosis. Hum Mol Genet 1997;6(6):927–33.
55 Gache Y, Baldeschi C, del Rio M et al. Construction of skin equivalents for gene therapy of recessive dystrophic epidermolysis bullosa. Hum Gene Ther 2004;15(10):921–33.
56 Gagnoux-Palacios L, Vailly J, Durand-Clement M, Wagner E, Ortonne JP, Meneguzzi G. Functional re-expression of laminin-5 in laminin-gamma2-deficient human keratinocytes modifies cell morphology, motility, and adhesion. J Biol Chem 1996;271(31):18437–44.
57 Garlick JA, Katz AB, Fenjves ES, Taichman LB. Retrovirus-mediated transduction of cultured epidermal keratinocytes. J Invest Dermatol 1991;97(5):824–9.
58 Goto M, Sawamura D, Ito K et al. Fibroblasts show more potential as target cells than keratinocytes in COL7A1 gene therapy of dystrophic epidermolysis bullosa. J Invest Dermatol 2006;126(4):766–72.
59 Seitz CS, Giudice GJ, Balding SD, Marinkovich MP, Khavari PA. BP180 gene delivery in junctional epidermolysis bullosa. Gene Ther 1999;6(1):42–7.
60 Vailly J, Pulkkinen L, Miquel C et al. Identification of a homozygous one-basepair deletion in exon 14 of the LAMB3 gene in a patient with Herlitz junctional epidermolysis bullosa and prenatal diagnosis in a family at risk for recurrence. J Invest Dermatol 1995;104(4):462–6.
61 Woodley DT, Krueger GG, Jorgensen CM et al. Normal and gene-corrected dystrophic epidermolysis bullosa fibroblasts alone can produce type VII collagen at the basement membrane zone. J Invest Dermatol 2003;121(5):1021–8.
62 Roth DA, Tawa NE Jr, O’Brien JM, Treco DA, Selden RF. Nonviral transfer of the gene encoding coagulation factor VIII in patients with severe hemophilia A. N Engl J Med 2001;344(23):1735–42.
63 Gallico GG 3rd, O’Connor NE, Compton CC, Kehinde O, Green H. Permanent coverage of large burn wounds with autologous cultured human epithelium. N Engl J Med 1984;311(7):448–51.
64 Llames SG, del Rio M, Larcher F et al. Human plasma as a dermal scaffold for the generation of a completely autologous bioengineered skin. Transplantation 2004;77(3):350–5.
65 Ronfard V, Rives JM, Neveux Y, Carsin H, Barrandon Y. Long-term regeneration of human epidermis on third degree burns transplanted with autologous cultured epithelium grown on a fibrin matrix. Transplantation 2000;70(11):1588–98.
66 Hengge UR, Walker PS, Vogel JC. Expression of naked DNA in human, pig, and mouse skin. J Clin Invest 1996;97(12):2911–16.
67 Cheng L, Ziegelhoffer PR, Yang NS. In vivo promoter activity and transgene expression in mammalian somatic tissues evaluated by using particle bombardment. Proc Natl Acad Sci USA 1993;90(10):4455–9.
68 Rakhmilevich AL, Turner J, Ford MJ et al. Gene gun-mediated skin transfection with interleukin 12 gene results in regression of established primary and metastatic murine tumors. Proc Natl Acad Sci USA 1996;93(13):6291–6.
69 Roos AK, Eriksson F, Walters DC, Pisa P, King AD. Optimization of skin electroporation in mice to increase tolerability of DNA vaccine delivery to patients. Mol Ther 2009;17(9):1637–42.
70 Zhang L, Nolan E, Kreitschitz S, Rabussay DP. Enhanced delivery of naked DNA to the skin by non-invasive in vivo electroporation. Biochim Biophys Acta 2002;1572(1):1–9.
71 Sawamura D, Ina S, Itai K et al. In vivo gene introduction into keratinocytes using jet injection. Gene Ther 1999;6(10):1785–7.
72 Ciernik IF, Krayenbuhl BH, Carbone DP. Puncture-mediated gene transfer to the skin. Hum Gene Ther 1996;7(8):893–9.
73 Hafeli UO, Mokhtari A, Liepmann D, Stoeber B. In vivo evaluation of a microneedle-based miniature syringe for intradermal drug delivery. Biomed Microdevices 2009;Apr 4 (epub ahead of print).
74 Yu WH, Kashani-Sabet M, Liggitt D, Moore D, Heath TD, Debs RJ. Topical gene delivery to murine skin. J Invest Dermatol 1999;112(3):370–5.
75 Smith FJ, Hickerson RP, Sayers JM et al. Development of therapeutic siRNAs for pachyonychia congenita. J Invest Dermatol 2008;128(1):50–8.
76 Alexander MY, Akhurst RJ. Liposome-medicated gene transfer and expression via the skin. Hum Mol Genet 1995;4(12):2279–85.
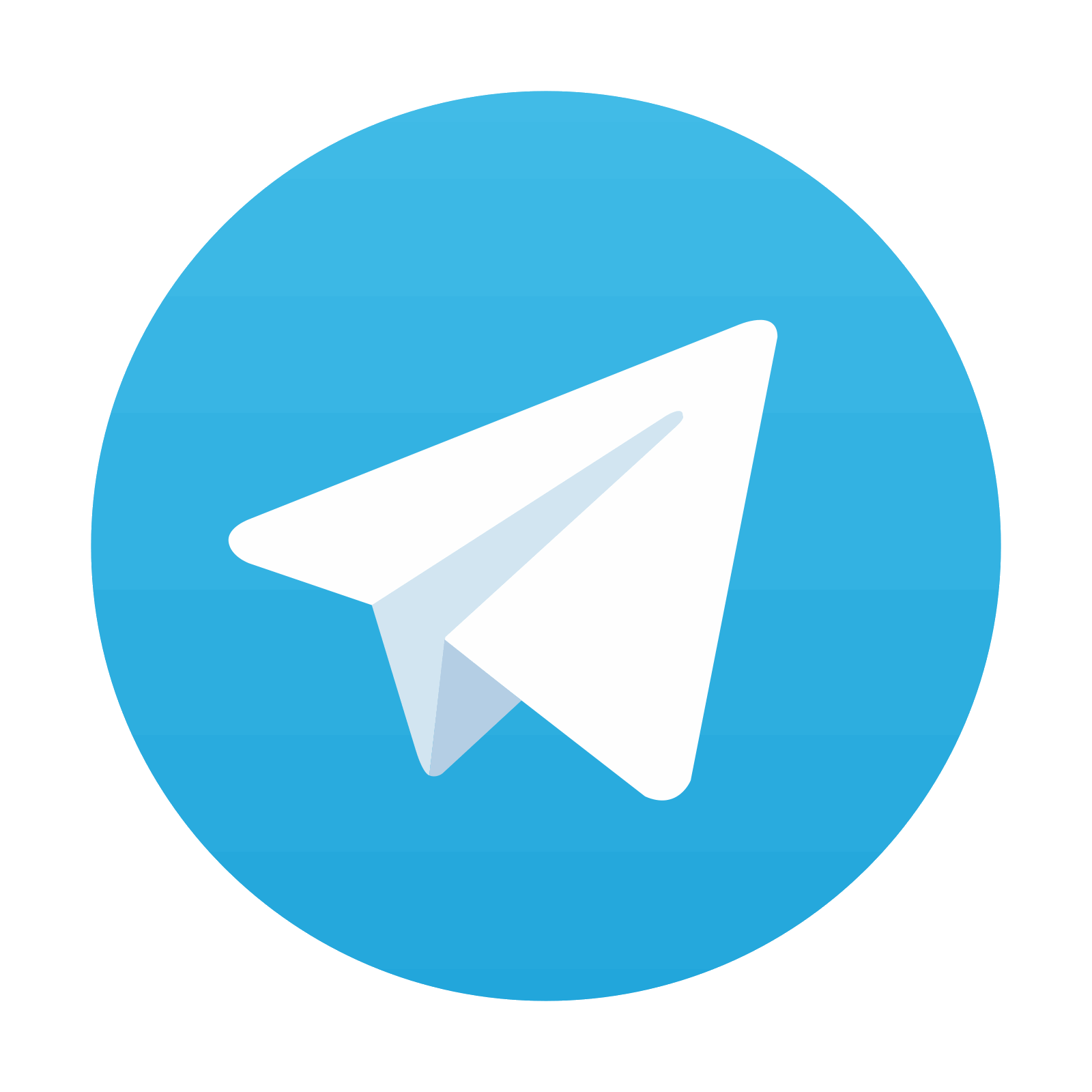
Stay updated, free articles. Join our Telegram channel
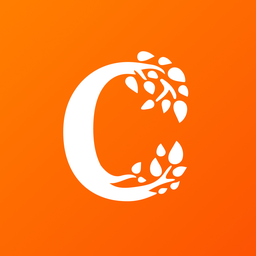
Full access? Get Clinical Tree
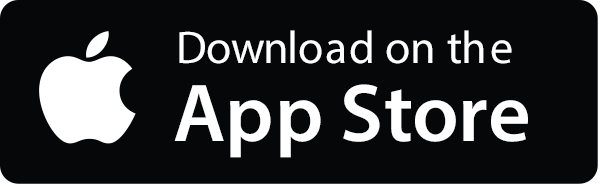
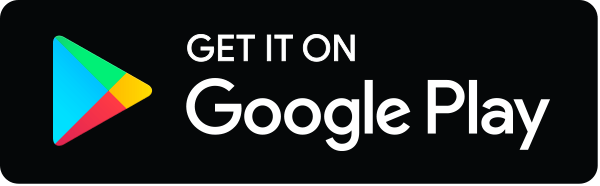