Fig. 1.1
Formation of embryonic stem cells within the inner mass of the blastocyst, days 0–6
Caution is obviously necessary under many aspects, first of all the need for an extensive animal experimentation before any clinical trial, in order to evaluate potential untoward effects; strategies have also been devised in order to avoid immunological rejection of transplanted cells [19]. A special case of production of undifferentiated ES cell lines is the so-called somatic cell nuclear transfer (SCNT). This is performed by replacing the nucleus of an unfertilized egg with a nucleus of a somatic cell of another subject. The egg is then stimulated to undergo embryonic development to the blastocyst stage; the inner mass is removed and embryonic stem cells are thus obtained and established as cell lines, which may undergo the process of differentiation [20].
It has to be noted that while the chromosomes of such ES cells are those from the donor, the egg retains a portion of DNA, the mitochondrial DNA, which resides in the cytoplasm. Therefore the developing embryo is not completely identical to the donor, unless the same person is donating both the egg and the nucleus.
A startling result was announced recently, namely, that it has been possible to form by SCNT early human embryos that can give rise to ES cells. The resulting stem cells are not normal, as they carry the genomes of both the adult cell and the oocyte: they have three copies of each chromosome instead of the usual two, and they do not survive beyond 4 weeks [21]. This result has raised a heated debate, because it involves creating a human embryo and then destroying it. Less credible is the fear that, if human nuclear transfer works in the lab, someone could eventually use it to clone a human being [22].
1.2.3 Induced Pluripotent Stem Cells (IPSCs)
In 2006, a team at Kyoto University in Japan reported that by adding a handful of genes to a skin cell, they could turn it into an induced pluripotent stem cell (IPSC), thus restoring the potential to develop new differentiated cells [23]. This was obtained by retroviral-mediated transduction of adult cells with four transcription factors, highly expressed in ES cells. After that report a remarkable progress followed, showing that IPSCs can be obtained by human adult cells and from a variety of tissues [24]. Besides the demonstration of a number of core transcription factors (OCT4, Mango, and Sox2), necessary to maintain pluripotency of ES cells, other factors have been identified, which can be used to induce reprogramming of the cell destiny in a number of somatic cells [25, 26].
IPSCs can be developed from patients affected by a number of disorders: the possibility therefore arises to produce by a combination of transcription factors patient-specific human induced stem cells, which can be used for in vitro disease modeling and regenerative medicine [27]. However, a number of problems have arisen about possible applications of human IPSCs in cell engineering and regenerative medicine: it is indeed demonstrated that a reprogramming process is not a genetic transformation but an epigenetic change, involving persistent somatic memory and aberrant DNA methylation [28]. It was actually shown that IPSCs derived by factor-based reprogramming of adult murine tissues still harbor residual DNA methylation signatures characteristic of their somatic tissue of origin, an epigenetic memory which favors their differentiation along lineages related to the donor cell, while restricting alternative cell fates [29]. Such memory of the donor tissue could be corrected by differentiation and serial reprogramming [30]. In addition, there is the possibility of a reactivation of the female inactivated X chromosome, an event observed in mouse fibroblasts, but apparently not in human IPSCs [31].
Another problem may arise from reprogramming genes, which may still be active in host cells so that foreign DNA remains in the host genome. It is thus necessary to avoid the integration of foreign sequences; to this aim, new techniques have been developed to remove the integrated DNA from the genome of IPSCs, making them safer for clinical use [32, 33].
An important development has been reported recently, namely, a way to obtain fast reprogramming of adult cells, using a potent transcriptional activator (MyoD), which can convert one cell type to another. Fusion of a MyoD fragment to one of the early transcription factors, full-length Oct4, might add to the powerful transactivation capacity of MyoD the target specificity of Oct4 [34]. This novel approach may produce a radical acceleration of IPSC generation.
From all such considerations, however, it appears that human IPSCs are not entirely comparable to embryonic stem (ES) cells as far as developmental capacity and genetic stability are concerned; it is therefore suggested that ES and IPSCs, due to their distinct features, may just complement each other for a variety of research and therapeutic applications [27].
1.2.4 Alternative Stem Cell Sources
It has been known for many years that the skin contains a rather vast variety of stem cells, including epidermal and mesenchymal stem cells (MSCs), melanocytic stem cells derived from the embryonic neural crest, as well as hematopoietic and endothelial precursors [35]. MSCs are of particular interest, as they exhibit a wide differentiation potential and, when transplanted in animals, show migratory capacity, being able to move to injury sites [36].
A distinct population of skin-derived progenitors has been isolated and characterized. These cells, named “skin-derived precursors (SKP),” can produce mesodermal and neural progeny [37]. More recent research has shown that SKP are neural crest precursors, located in a specific dermal niche and possessing a wide differentiation potential, which make them suitable for therapeutic use [38]. A latest example of how skin fibroblast-derived stem cells may be used in different areas is supplied by a report from a team at the Mayo Clinic, which has obtained induced pluripotent stem cells (IPSCs) from the skin of patients in end-stage kidney diseases. Such IPSCs may be used not only for the study of renal disease pathogenesis but also as autologous elements, for a trial of new kidney replacement therapy [39].
Among the adult cells which have been shown capable of reverting to a condition of IPSCs by appropriate in vitro treatment, a very interesting place is occupied by mature adipose cells [40]. Their advantage is that they can be obtained in large amounts with minimal effort and can apparently maintain a kind of “staminal” characteristics during passage in culture, so that they may be eventually employed in attempts of tissue regeneration [41]. It has been shown indeed that purified adipose stem cells express high level of markers of staminality like CD49d, CD44, CD90, CD105, CD13, and CD71 and that these markers are maintained at high level for at least 3 months and 7 passages of in vitro culture [42]. Progenitor, endothelial, and mesenchymal stem cells derived from adipose tissues are receiving great attention in view of reconstructive surgery applications; they also may be considered for cell therapy in a number of disease conditions, including those affecting the bone, cartilage, muscle, liver, kidney, cardiac, neural, and pancreatic tissues [43].
An interesting source of stem cells has received attention recently, namely, the amniotic fluid. Amniotic fluid-derived stem (AFS) cells are easily collected, have a high renewal capacity, and can differentiate into all tissues derived from all embryonic layers [44]. Of special interest is their application in orthopedic surgery, where they can be used in combination with titanium plates and screws to obtain satisfactory osteosynthesis [45]. It has been recently confirmed that AFS cells can be maintained for over 250 population doublings while preserving their telomere length and a normal karyotype. Therefore, many more uses for AFS cells are now considered in regenerative medicine [46].
Another source, connected to the amniotic fluid, is represented by placental stem cells, which are also available in considerable amount and show differentiation potential toward all three layers of germ cells [47]. Their possible clinical applications are being investigated.
1.3 Stem Cell Treatment
The last decade has seen a tremendous increase in both the fields and types of stem cell applications for the treatment of a wide variety of pathological conditions. The author shall, therefore, give in this survey a general outline of an ever-expanding area. It is anyway proper to start mentioning the field of the earliest and now historical trials of stem cell therapy, namely, hematopoietic cell transplantation (HST). This was successfully studied and performed by a pioneering team in Seattle [48], but a number of scientists in many countries have contributed to the development of such technique, particularly in identifying all types of blood stem cells and their different sources. For instance, it has been obtained a successful use of stem cells from peripheral blood, with the added bonus of their mobilization before collection [49].
Stem cells from the umbilical vein, or cord blood (CB) stem cells, have also been found in considerable amount and successfully used for transplantation. It was found that CB stem cells can be amplified in culture, where they are capable of extensive self-renewal [50, 51].
Cord blood banks have been set up, and strict rules have been established for a correct collection and preservation of samples [52]. Storage can be either in private banks, where CB stem cells can be used as autologous or allogeneic transplants for the infant donor or related family members, or in public institution, where samples are not available to the public; these banks however are now part of registries for a general search of donor sources [53].
The advantages of cord blood stem cell therapy are of course the access to cord blood banks all over the world, a low risk of transmitting infections, good tolerance of human leukocyte antigen (HLA) disparity, lower incidence of severe graft-versus-host disease (GVHD), and lasting immune recovery. It has also been stressed that, besides hematopoietic stem cells, cord blood contains endothelial cells, mesenchymal stromal cells, T-regulatory cells, dendritic cells, and natural killer cells and that these cell types are becoming an important field of investigation, aiming at new treatment options [54].
The applications of HST in clinical hematology have actually increased enormously from the primitive area of blood malignancies to red cell anomalies like severe hemoglobinopathies and a number of other disorders [55]. The process of HST involves of course a wide range of questions, like the choice of the donor, who can be either the patient (autologous HST) or a related or unrelated person (allogeneic HST). Of primary importance are also the conditioning regimens to ablate the unwanted marrow population.
As far as Hbpathies are concerned, HST has been mainly practiced in conditions like β-thalassemia (β-Thal) and sickle-cell disease (SCD), which are presently the most common inherited diseases worldwide. Such disorders can occur in a different variety of forms, most of which can be usually treated in a conservative manner. However, β-Thal major and severe SCD are life-threatening conditions, and for them HST offers the only chance of a cure. A general consensus considers suitable for transplant thalassemic patients under 17 years of age, who are lucky to have an HLA-identical sibling donor, while for SCD patients transplantation is generally delayed until evidence of a very severe disease, as in the occurrence of a stroke [56].
As for conditioning regimens, there has been some favor for minimally toxic and nonablative treatments, which however may produce only a transient engraftment. At present, conditioning regimens include eradication of the hematopoietic system by administration of a high dose of busulfan and suppression of the immune system by administration of cyclophosphamide [57]. To give an idea of the incredible potential of hematopoietic transplants, it is sufficient to remember a repopulation experiment of multi-lineage engraftment in mice from just a single bone marrow transplanted cell, as reported by Krause et al. [58].
A new promising approach has been recently the attempt to combine cell and gene therapy by globin gene transfer for both thalassemia and SCD. The therapeutic efficacy of this procedure has been established in several mouse models of β-Thal and Sickle Cell Disease; safety could be obtained using lentiviral vectors, which provide therapeutic transgene expression, restricted to late stages of differentiation within the erythroid lineage [59]. Of great interest was the report of an adult patient with severe β-thalassemia, dependent on monthly transfusions since early childhood, who has become transfusion-independent following lentiviral β-globin gene transfer, although a potentially oncogenic clone had appeared [60].
Hematopoietic stem cell transplantation has also been performed in a very severe group of anomalies, namely, primary immune deficiencies, ranging from severe combined immune deficiency (SCID) to other related conditions. Survival in both SCID and non-SCID syndromes was of course better with geno-identical donors, around 90 %, than in mismatched or unrelated donors, around 66 % [61]. HST using autologous stem cells, in conjunction with gene therapy, has been employed in amino-deaminase deficiency (ADA), a fatal disorder, obtaining significant benefits, with immune reconstitution in some patients and no more requirement for enzyme replacement therapy [62].
Recent research has shown that the use of patient-derived induced pluripotent stem cells (IPSCs) may overcome some of the inherent limitations of the previous procedures. New approaches have also been adopted to avoid the risk of leukemic induction due to insertional mutagenesis: target-specific enzymes are thus introduced to facilitate gene correction or gene insertion into secure gene locations, or “safe harbors” [63].
An interesting application has been transplantation in utero (IUHST), taking advantage of fetal immunological immaturity, which allows to omit conditioning regimens before transplantation: this procedure has been performed with good success in diverse conditions like Hbpathies and immune deficiencies [64]. Moreover, experimental progress has been made using IUHST to induce donor-specific tolerance and thus facilitate a relatively nontoxic postnatal HST: a strategy which promises a vast field of clinical application of IUHST [65]. In an interesting development, it was found that engraftment may be hampered in mice by maternal T cells, and it is therefore suggested that the clinical success of IUHST may be improved by transplanting cells matched to the mother [66].
As far as other blood disorders treated with HST, mention has to be made of Fanconi anemia (FA), a severe congenital form of pediatric aplastic anemia, with frequent evolution into myelodysplasia syndrome (MDS) and/or acute myeloid leukemia (AML) with a specific pattern of somatic chromosomal lesions. However, a relevant proportion of FA patients can now be successfully cured by HST, even in the absence of an HLA-identical sibling, especially if the conditioning regimen includes fludarabine [67].
1.3.1 Neurological Disorders
Neural stem cells (NSCs) can be isolated and grown in culture as clusters called “microspheres,” following stimulation by appropriate factors. They also show a broad differentiation potential, which may act not only along the neural lineage to produce adult neurons but also along other so far unsuspected directions [68]. It was also found in early investigation not only that such property may be used as a replacement of lost cells but that NSCs can promote proliferation and reorganization of endogenous stem cells and tissues [69].
The advent of induced pluripotent stem cells (IPSCs) has prompted of course new experimentation in order to transform them into neural progenitors, insert missing genes, and proceed to various forms of gene therapy. The therapeutic potential of stem cell treatment in neurology is so wide that we can only mention some items. Disease-specific IPSCs were derived from patients of several neurodegenerative diseases, including Parkinson’s disease, Alzheimer’s disease, amyotrophic lateral sclerosis, and spinal muscular atrophy: neurons differentiated from these IPSCs reproduce the actual pathological picture and thus allow in the first place a test for drug activity, but they could also be used as a model for cell replacement [70].
A good example is the case of Parkinson’s disease (PD), where gene therapy is aiming to provide the missing chemical, dopamine, and others to the affected cells of PD patients. Clinical trials include the attempts to insert useful genes for production, storage, and uptake of dopamine by the dopaminergic pathways of the basal ganglia in the brain [71]. Researchers using human IPSCs derived from PD patients determined indeed that transplanted differentiated cells were able to survive, engraft, and differentiate into dopaminergic neurons while favorable functional effects were also noted. Long-term survival of the transplanted cells was also detected, and no evidence of PD pathology, such as inclusion body formation, was observed in the transplanted neurons [72].
A special case is a rare disease, X-linked adrenoleukodystrophy (ALD), in which gene therapy using autologous transplanted hematopoietic cells has actually been performed with success. This is a fatal, congenital, demyelinating disease of the nervous system, caused by a gene mutation of the ALD protein that protects the nerve myelin envelope. Two patients receiving lentivirus-transduced stem cells obtained an arrest of progressive cerebral damage and a significant clinical benefit [73].
Important developments have been recently the successful attempts to generate autologous neurons directly from dermal fibroblasts using cellular extracts, without the need of passing through a stage of pluripotency. This procedure, which has the great advantage of avoiding genetic manipulation with the relative risks, is also presently applied to other cell types, like cardiomyocytes [74].
1.3.2 Cardiac Failure
The presently available remedy for severe heart failure, namely, heart transplantation, still has limited application, due to donor scarcity and problems of rejection. Therefore, an increasing amount of research is directed to the possibility of cardiac tissue regeneration, trying a wide variety of sources from embryonic stem cells to bone marrow mononuclears and even adipose tissue stem cells [75]. Some direct progenitors of cardiac tissue were actually found in the epicardium itself, where multipotent stem cells have been shown capable of giving rise to all three lineages of the heart, namely, cardiomyocytes, smooth muscle, and endothelium [76].
Numerous experiments were performed using a variety of these progenitor cells in animal models and also in a few clinical trials, with some encouraging but short-term results [77]. However, recent efforts to promote cardiomyocyte regeneration have recorded a remarkable progress, with the demonstration that cardiac fibroblasts can be reprogrammed in vitro to new differentiated cells, like cardiomyocytes, by appropriate cocktails of transcription factors involved in heart development [78]. Two teams (from San Francisco and Dallas), after inducing myocardial infarction in experimental mice, inserted by retroviral transduction [79] the genes encoding four transcription factors (GATA4, MEF2C, Tbx5, and HAND2) into the injured area, in order to produce cardiomyocyte-like cells (it is actually known that retroviruses can only insert genes into dividing cells, like scar-forming fibroblasts, but not into cardiomyocytes, which do not divide). After a few weeks, the projected goal was brilliantly achieved, as the new cells were actively proliferating and helping to improve the cardiac function [80, 81]. It is however likely that original cardiomyocyte progenitors (stemlike cells) may have a higher proliferation rate and would therefore be more effective in obtaining cardiomyocyte regeneration, an open question so far.
1.3.3 Cell Therapy for Diabetes
When pancreatic β-cells are destroyed via an autoimmune reaction in type 1 diabetes (T1D), islet transplantation has been widely tried with variable success. However, this is a rather impractical procedure, due to the limited number of cadaveric islet donors and immunological problems [82].
An obvious alternative is the use of pluripotent stem cells, which provide an unlimited cell source to generate new β-cells; furthermore, the advent of induced pluripotent stem cells (IPSCs) from patients has produced a powerful tool for autologous cell replacement therapy, disease modeling, and drug screening for both types of diabetes [83].
In order to generate functional insulin-producing cells, embryonic stem cells must first differentiate to endoderm and pancreatic endocrine progenitors. In this process, activin A (a member of the transforming growth factor beta superfamily) has shown a remarkable activity, given the proper concentration, culture conditions, and time of application; other factors like serum components or contamination by feeder cells as well as differentiation and proliferation factors play a critical role in such sequence of events [84].
An interesting protocol, carried out in a serum-free system, has been described, by which human embryonic (ES) cells are induced to differentiate into functional insulin-producing cells: activin A is used in the initial stage to induce definitive endoderm lineage differentiation from human ES cells, while all-trans retinoic acid (RA) is then utilized to promote pancreatic differentiation. Final maturation is induced by fibroblast growth factors and nicotinamide, so that the differentiated cells express islet specific markers [85]. Further investigation is anyway necessary to implement strategies for cell therapy in the different clinical types of diabetes mellitus: in particular, more protocols are required to obtain a reproducible generation of insulin-making cells in culture [82].
1.3.4 Diseases of the Skin and Its Appendages
Skin transplants have a rather long history, if we believe an illustrious character like Winston Churchill, who, as an officer during the war in Sudan in 1898, donated a portion of his skin to a wounded friend and described the operation as very successful [86]. There is indeed a population of stem cells in the epidermis, which is capable of self-renewal and differentiation and thus capable of providing a fast renewal of that tissue [87].
It has thus been possible in the past to apply different types of skin grafts in order to treat a variety of conditions, like skin wounds, venous ulcers, and burns. In recent years, however, growing attention has been devoted to various forms of engineered stem cells, to obtain repair of disrupted skin and appropriate regeneration [88]. Stem cell-based skin engineering in conjunction with gene recombination, in which the stem cells act as both the seed cells and the vehicle for gene delivery to the wound site, represents the most attractive model for a regenerative strategy of wound therapy [89].
In addition to the indications mentioned before, severe inherited skin disorders represent a very challenging area for stem cell replacement, combined with gene therapy. An example of a clearly identifiable candidate genetic disease is epidermolysis bullosa, which is a group of heritable devastating disorders, characterized by fragility of the skin and mucous membranes. A successful transplant with genetically corrected autologous stem cell was first performed in 2006 [90], but it was pointed out that its effects were limited to the area of application and still with risks of gene augmentation due to traditional retroviral constructs: all this prompts a search for a systemic and safer approach [91].
A most innovative attempt to combine stem cell lines with a suitable material has been recently introduced, utilizing native spider silk woven on steel frames, which can provide a good matrix for 3-dimensional skin cell culturing. Fibroblasts and keratinocytes cell lines, guided by the silk fibers, proliferate and reach confluence in a few days, initiating rapid skin regeneration [92].
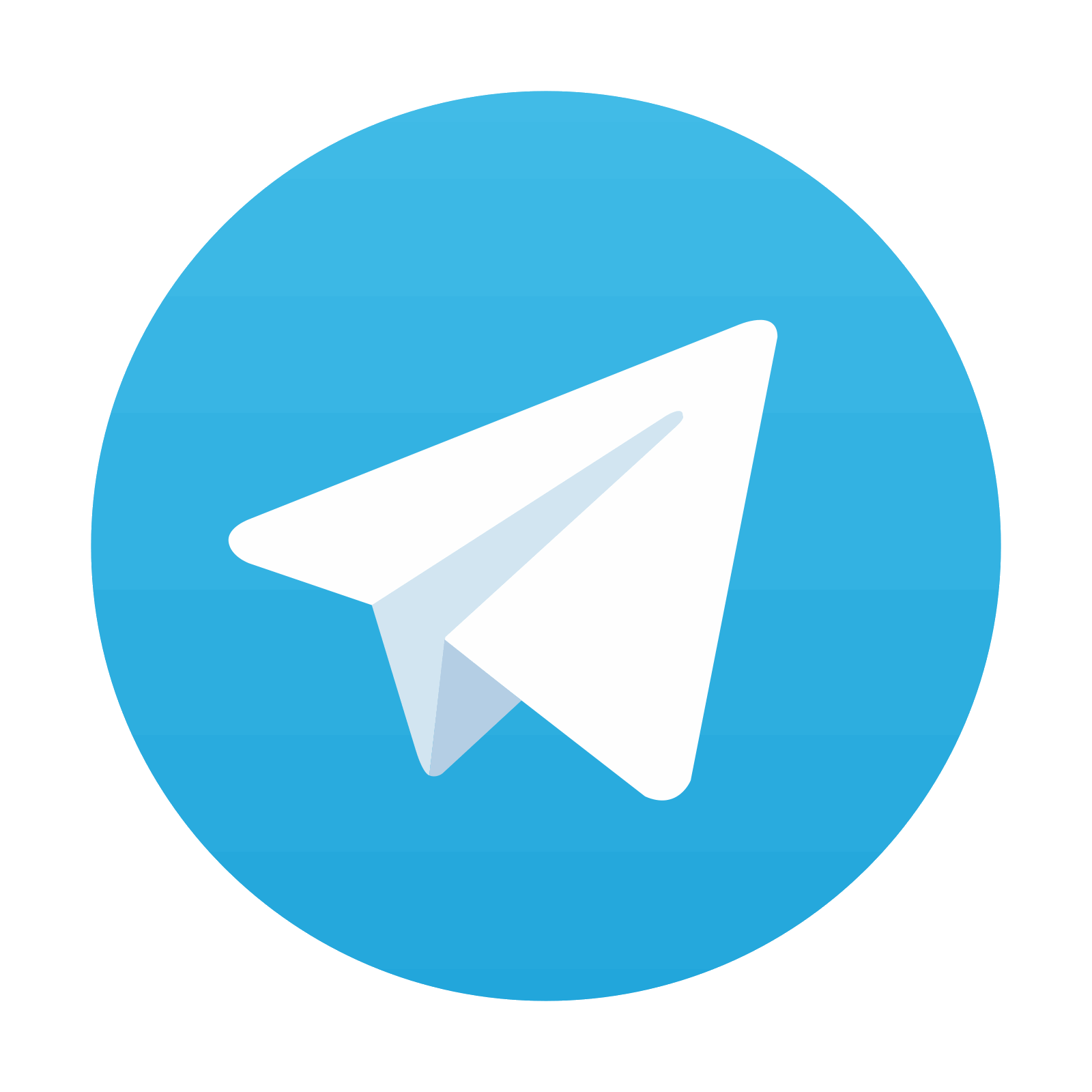
Stay updated, free articles. Join our Telegram channel
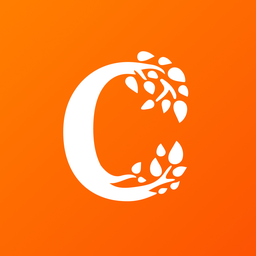
Full access? Get Clinical Tree
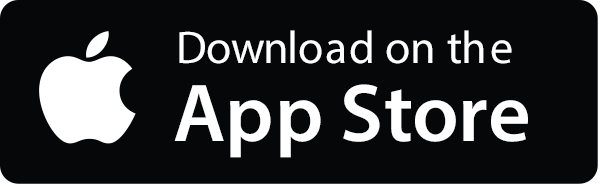
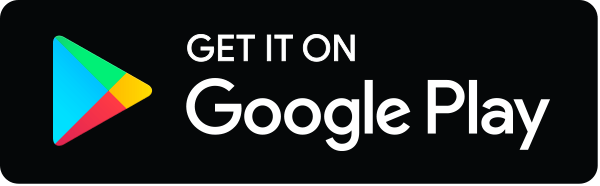