© Springer-Verlag Berlin Heidelberg 2017
Nina Dragicevic and Howard I. Maibach (eds.)Percutaneous Penetration Enhancers Physical Methods in Penetration Enhancementhttps://doi.org/10.1007/978-3-662-53273-7_11. Sonophoresis: Ultrasound-Mediated Transdermal Drug Delivery
(1)
Department of Chemical Engineering, University of California, Santa Barbara, CA 93106, USA
Keywords
UltrasoundLow frequencyMechanismClinicalOverview1.1 Introduction
Transdermal drug delivery offers several advantages over traditional drug delivery systems such as oral delivery and injections (Prausnitz et al. 2004). In spite of its advantages, transdermal drug delivery suffers from the severe limitation that the permeability of the skin to all but a few small and hydrophobic molecules is very low. Therefore, it is difficult to deliver drugs across the skin at a therapeutically relevant rate. Only a handful of low-molecular-weight drugs are clinically administered by this route today. Overcoming skin’s barrier properties safely and reversibly is a fundamental problem that drives innovation in the field of transdermal delivery.
1.2 Ultrasound and Sonophoresis
Ultrasound is used in various medical therapies (Mitragotri 2005), including lithotripsy (Coleman and Saunders 1993), hyperthermia (Diederich and Hynnen 1999), thrombolysis (Alexandrov 2002), lipoplasty (Goes and Landecker 2002), wound healing (Speed 2001), fracture healing (Hadjiargyrou et al. 1998), and drug delivery (Kost et al. 1989; Mitragotri et al. 1995a; Miller and Quddus 2000; Guzman et al. 2001; Kwok et al. 2001; Tezel et al. 2001b; Unger et al. 2001; Linder 2002; Nelson et al. 2002; Price and Kaul 2002; Sundaram et al. 2002; Tang et al. 2002a, b; Wu et al. 2002). Ultrasound under various conditions has been used to enhance transdermal transport of drugs including macromolecules (Griffin and Touchstone 1963, 1968, 1972; Cameroy 1966; Griffin et al. 1967; Kleinkort and Wood 1975; Quillen 1980; Benson et al. 1988; Benson et al. 1989; Williams 1990; Benson et al. 1991; Ciccone et al. 1991; Tachibana and Tachibana 1991; Bommannan et al. 1992a, b; Tachibana 1992; Byl et al. 1993; Kost and Langer 1993; Machluf and Kost 1993; Tachibana and Tachibana 1993; Menon et al. 1994; Mitragotri et al. 1995a,1996a, b, 1997, 2000a, d, Mitragotri 2000; Mitragotri and Kost 2000a, b; Johnson et al. 1996; Kost et al. 1996; Kost et al. 1999; Kost et al. 2000; Le et al. 2000; Tang et al. 2001; Tezel et al. 2001a, b; Joshi and Raje 2002; Machet and Boucaud 2002; Tang et al. 2002a, b; Terahara et al. 2002a, b; Tezel et al. 2002a, b; Weimann and Wu 2002; Alvarez-Roman et al. 2003; Merrino et al. 2003; Merriono et al. 2003; Tezel et al. 2003). This type of enhancement is termed sonophoresis, indicating the enhanced transport of molecules under the influence of ultrasound. Ultrasound at various frequencies in the range of 20 kHz–16 MHz has been used to enhance skin permeability. However, transdermal transport enhancement induced by low-frequency ultrasound (f < 100 kHz) has been found to be more significant than that induced by high-frequency ultrasound (Mitragotri et al. 1995a, b; Tezel et al. 2001a, b; Boucaud et al. 2002).
The first published report on sonophoresis dates back to 1950s. Fellinger and Schmidt (Fellinger and Schmidt 1954) reported successful treatment of polyarthritis of the hand’s digital joints using hydrocortisone ointment with sonophoresis. It was subsequently shown that hydrocortisone injection combined with ultrasound massage yielded better outcome compared to simple hydrocortisone injections for bursitis treatment (Coodley 1960). Cameroy (1966) reported success using carbocaine sonophoresis for closed Colles fractures. Griffin et al. showed improved treatment of elbow epicondylitis, bicipital tendonitis, shoulder osteoarthritis, shoulder bursitis, and knee osteoarthritis by combined application of hydrocortisone and ultrasound (Griffin 1966; Griffin et al. 1967; Griffin and Touchstone 1968, 1972). Improved dermal penetration of local anesthetics was also demonstrated using ultrasound (Moll 1979; McElnay et al. 1985; Benson et al. 1988).
Studies demonstrated that ultrasound enhanced the percutaneous absorption of nicotinate esters by disordering the lipids in the stratum corneum (SC) (Benson et al. 1991; McElnay et al. 1993). Similar conclusions were reached by Hofman and Moll (Hofman and Moll 1993) who studied the percutaneous absorption of benzyl nicotinate. While several investigators reported positive effect of ultrasound on drug permeation, lack of an effect of ultrasound on skin permeation was also reported in certain cases. For example, Williams reported no detectable effect of 1.1-MHz ultrasound on the rate of penetration of three anesthetic preparations through human skin (Williams 1990).
Levy et al. (1989) showed that 3–5 min of ultrasound exposure (1 MHz, 1.5 W/cm2) increased transdermal permeation of mannitol and physostigmine across hairless rat skin in vivo by up to 15-fold. They also reported that the lag time typically associated with transdermal drug delivery was nearly completely eliminated after exposure to ultrasound. Mitragotri et al. reported in vitro permeation enhancement of several low-molecular-weight drugs under the same ultrasound conditions (Mitragotri et al. 1995b).
Bommannan et al. (1992a, b) hypothesized that since the absorption coefficient of the skin varies directly with the ultrasound frequency, high-frequency ultrasound energy would concentrate more in the epidermis, thus leading to higher enhancements. In order to assess this hypothesis, they studied the effect of high-frequency ultrasound (2–16 MHz) on permeability of salicylic acid (dissolved in a gel) through hairless guinea pig skin in vivo. They found that a 20-min application of ultrasound (0.2 W/cm2) at a frequency of 2 MHz did not significantly enhance the amount of salicylic acid penetrating the skin. However, 10-MHz ultrasound under otherwise the same conditions resulted in a fourfold increase, and 16-MHz ultrasound resulted in about a 2.5-fold increase in transdermal salicylic acid transport (Bommannan et al. 1992a, b).
1.3 Low-Frequency Sonophoresis
Low-frequency sonophoresis has been a topic of extensive research only in the last 20 years. Tachibana et al. (Tachibana and Tachibana 1991, 1993; Tachibana 1992) reported that application of low-frequency ultrasound (48 kHz) enhanced transdermal transport of lidocaine and insulin across hairless rat skin in vivo. They found that the blood glucose level of a hairless rat immersed in a beaker filled with insulin solution (20 U/ml) and placed in an ultrasound bath (48 kHz, 5000 Pa) decreased by 50 % in 240 min (Tachibana and Tachibana 1991). They also showed that application of ultrasound under similar conditions prolonged the anesthetic effect of transdermally administrated lidocaine in hairless rats (Tachibana and Tachibana 1993) and enhanced transdermal insulin transport in rabbits. Mitragotri et al. (1995a, b, 1996a, b) showed that application of ultrasound at even lower frequencies (20 kHz) enhances transdermal transport of various low-molecular-weight drugs including corticosterone and high-molecular-weight proteins such as insulin, γ-interferon, and erythropoietin across the human skin in vitro. Mitragotri et al. compared the enhancement ratios induced by therapeutic ultrasound (1 MHz) and low-frequency ultrasound (20 kHz) for four permeants, butanol, corticosterone, salicylic acid, and sucrose. They found that the enhancement induced by low-frequency ultrasound is up to 1000-fold higher than that induced by therapeutic ultrasound (Mitragotri et al. 1996a, b). Low-frequency sonophoresis-mediated permeation of a number of small molecules including ketoprofen, lidocaine, ascorbic acid, caffeine, cortisol, fentanyl, and estradiol across the skin has been tested. A detailed list of molecules tested under low-frequency sonophoresis is provided in Ref. Polat et al. (2011).
Low-frequency sonophoresis can be classified into two categories: simultaneous sonophoresis and pretreatment sonophoresis. Simultaneous sonophoresis corresponds to a simultaneous application of drug and ultrasound to the skin. This was the first mode in which low-frequency sonophoresis was shown to be effective. This method enhances transdermal transport in two ways: (i) enhanced diffusion through structural alterations of the skin and (ii) convection induced by ultrasound (Tang et al. 2002a, b). Transdermal transport enhancement induced by this type of sonophoresis decreases after ultrasound is turned off (Mitragotri et al. 2000a, b, c). Although this method can be used to achieve a temporal control over skin permeability, it requires that patients use a wearable ultrasound device for drug delivery. In pretreatment sonophoresis, a short application of ultrasound is used to permeabilize the skin prior to drug delivery. The skin remains in a state of high permeability for several hours. Drugs can be delivered through permeabilized skin during this period. In this approach, the patient does not need to wear the ultrasound device. Pretreatment sonophoresis has been tested in the clinic (Becker et al. 2005; Gupta and Prausnitz 2009; Kim do et al. 2012).
1.4 Low-Frequency Sonophoresis: Choice of Parameters
The enhancement induced by low-frequency sonophoresis is determined by four main ultrasound parameters, frequency, intensity, duty cycle, and application time. A detailed investigation of the dependence of permeability enhancement on frequency and intensity in the low-frequency regime (20 kHz < f <100 kHz) has been reported by Tezel et al. (2001b). At each frequency, there exists an intensity below which no detectable enhancement is observed. This intensity is referred to as the threshold intensity. Once the intensity exceeds this threshold, the enhancement increases strongly with the intensity until another threshold intensity, referred to as the decoupling intensity, is reached. Beyond this intensity, the enhancement does not increase with further increase in intensity due to acoustic decoupling. The threshold intensity for porcine skin increased from about 0.11 W/cm2 at 19.6 kHz to more than 2 W/cm2 at 93.4 kHz. At a given intensity, the enhancement decreased with increasing ultrasound frequency.
The dependence of enhancement on intensity, duty cycle, and application time can be combined into a single parameter, total acoustic energy fluence delivered from the transducer, defined as E = It, where I is the ultrasound intensity (W/cm2) during each pulse and t is the total “on time” (seconds). As a general trend, no significant enhancement is observed until a threshold energy fluence dose is reached. The threshold energy fluence increases with increasing frequency. Specifically, the threshold energy fluence dose increased by about 130-fold as the frequency increased from 19.6 to 93.4 kHz. The dependence of enhancement on energy fluence after the threshold is different for different frequencies. For extremely high-energy doses (say 104 J/cm2), the enhancement induced by all the frequencies is comparable. However, for lower energy fluence doses, the differences between various different frequencies are significant and the choice of frequency may affect the effectiveness of sonophoresis.
In addition to frequency and energy fluence, sonophoretic enhancement also depends on additional parameters including the distance between the transducer and the skin (Terahara et al. 2002a, b), gas concentration in the donor compartment (Terahara et al. 2002a, b), and simultaneous exposure to other ultrasound frequencies (Schoellhammer et al. 2012).
1.5 Mechanism of Low-Frequency Sonophoresis
Significant attention has been devoted to understand the mechanisms of low-frequency sonophoresis (Mitragotri et al. 1995b; Tang et al. 2002b; Merino et al. 2003; Tezel and Mitragotri 2003). Acoustic cavitation, the formation and collapse of gaseous cavities, has been shown to be responsible for low-frequency sonophoresis (Tang et al. 2002b; Tezel and Mitragotri 2003). Cavitation originates from the growth of cavitation nuclei in response to oscillatory pressure fluctuations during cavitation. During low-frequency sonophoresis, cavitation is predominantly induced in the coupling medium (the liquid present between the ultrasound transducer and the skin (Tezel et al. 2002a). The maximum radius reached by free cavitating bubbles is related to the frequency and acoustic pressure amplitude. Under the conditions used for low-frequency sonophoresis (f ~ 20–100 kHz and pressure amplitudes ~1–2.4 bar), the maximum bubble radius is estimated to be between 10 and 100 μm.
Two types of cavitation, stable or inertial, have been evaluated for their role in sonophoresis. Stable cavitation corresponds to periodic growth and oscillations of bubbles, while inertial cavitation corresponds to violent growth and collapse of cavitation bubbles (Suslick 1989). Using acoustic spectroscopy, stable as well as inertial cavitation has been quantified (Tang et al. 2002b; Tezel et al. 2002a). The overall dependence of inertial cavitation on ultrasound intensity was found to be similar to that of conductivity enhancement (Tang et al. 2002b; Tezel et al. 2002a). Specifically, ultrasound intensity above the threshold value is required before inception of inertial cavitation is observed. This threshold corresponds to minimum pressure amplitude required to induce rapid growth and collapse of cavitation nuclei. Beyond this threshold, white noise (indicator of inertial cavitation) increased linearly with ultrasound intensity, although at any given intensity, inertial cavitation activity decreased rapidly with ultrasound frequency (Tezel et al. 2002a). The threshold intensity for the occurrence of inertial cavitation increased with increasing ultrasound frequency. This dependence reflects the fact that growth of cavitation bubbles becomes increasingly difficult with increasing ultrasound frequency. Tezel et al. showed that regardless of the intensity and frequency, skin conductivity enhancement correlated universally with a measure proportional to the total acoustic energy fluence (Tezel et al. 2002a). These data suggested a strong role played by inertial cavitation in low-frequency sonophoresis.
Inertial cavitation occurs in the bulk coupling medium as well as near the skin surface. Inertial cavitation at both locations may potentially be responsible of conductivity enhancement. Three mechanisms by which inertial cavitation events might enhance SC permeability were proposed (Tezel and Mitragotri 2003). These include bubbles that collapse symmetrically and emit a shock wave, which can disrupt the SC lipid bilayers, and those that collapse asymmetrically and give rise to acoustic microjets that impact the SC. Impact of microjets may also be responsible for SC lipid bilayer disruption. Microjets resulting from collapsing bubbles near the SC surface may also potentially penetrate into the SC and disrupt the structure.
Inertial cavitation in the vicinity of a surface is fundamentally different from that away from the surface. Specifically, collapse of spherical cavitation bubbles in the bulk solution is symmetric and results in the formation of a shock wave. This shock wave can potentially disrupt the structure of the lipid bilayers. However, the amplitude of the shock wave decreases rapidly with the distance. Cavitation bubbles migrate under the influence of ultrasound field toward the boundary and collapse near the boundary depending on its proximity to the surface (Naude and Ellis 1961). The collapse of cavitation bubbles near the boundary is asymmetric due to the difference in the surrounding conditions giving rise to the formation of a liquid microjet directed toward the surface. The diameter of the microjet is much smaller than that of the maximum bubble radius and the speeds are expected in the range of 50–180 m/s (Benjamin and Ellis 1966; Plesset and Chapman 1971; Lauterborn and Bolle 1975).
Tezel et al. evaluated the effect of spherical collapses as well as microjets on skin permeability enhancement (Tezel and Mitragotri 2003). They concluded that both types of cavitation events may be responsible for sonophoresis and about 10 collapses/s/cm2 in the form of spherical collapses or microjets near the surface of the stratum corneum may explain experimentally observed conductivity enhancements.
1.6 Permeation Pathways of Low-Frequency Sonophoresis
Significant effort has been focused on understanding the mechanisms of low-frequency sonophoresis and permeation pathways through the skin. Cavitation-induced disruption of SC lipid bilayers due to bubble-induced shock waves or microjet impact may enhance skin permeability by at least two mechanisms. First, a moderate level of disruption decreases the structural order of lipid bilayers and increases solute diffusion coefficient (Mitragotri 2001). At a higher level of disruption, lipid bilayers may lose structural integrity and facilitate penetration of the coupling medium into the SC. Since many sonophoresis experiments reported in the literature are performed using coupling media comprising aqueous solutions of surfactants, disruption of SC lipid bilayers enhances incorporation of surfactants into lipid bilayers. Incorporation of excessive water and surfactants further promotes bilayer disruption, thereby opening pathways for solute permeation (Walters 1990; Black 1993). Alvarez-Roman et al. reported that lipid extraction also plays a role in low-frequency sonophoresis (Alvarez-Roman et al. 2003). Ultrasound has also been shown to induce convective flow across the skin. Morimoto et al. reported that 41-kHz ultrasound has the potential to induce convective solvent flow to increase the skin permeation of hydrophilic calcein in excised hairless rat skin (Morimoto et al. 2005). Similar conclusions have also been reached by Tang et al. (2001).
The effects of ultrasound-induced cavitation on the skin have been shown to be highly heterogeneous in nature (Tezel et al. 2001b, 2002a, b; Alvarez-Roman et al. 2003; Merino et al. 2003; Kushner et al. 2004; Paliwal et al. 2006; Kushner et al. 2007, Kushner et al. 2008), leading to localized regions of high permeability. These highly permeabilized regions were labeled localized transport regions (LTRs). Using calcein as a model permeant, Kushner et al. demonstrated that the localized transport regions (LTRs) are approximately 80-fold more permeable than the surrounding regions of the skin (the non-LTRs) (Kushner et al. 2004). The difference in the enhancements of the LTRs and the non-LTRs was confirmed using skin electrical resistivity measurements. The skin electrical resistivity in the LTRs was found to be approximately 30-fold lower than in the non-LTRs. Transport was enhanced even in the non-LTR region. Specifically, the skin electrical resistivity of the non-LTRs was found to be approximately 170-fold lower than that of untreated skin, while the skin electrical resistivity of the LTRs was found to be approximately 5000-fold lower than that of untreated skin. Using mathematical models, the authors showed that the porosity values of the LTRs were three- to eightfold higher than those of the non-LTRs, while only a small difference in the tortuosity values of the LTRs and of the non-LTRs was observed. These results suggest that the difference in the permeabilities of the LTRs and non-LTRs is due to the creation of more aqueous pathways in the LTRs than in the non-LTRs (Kushner et al. 2008). In a subsequent investigation using two-photon microscopy, the same authors confirmed the presence of LTRs and reported on transcellular pathways in the LTRs (Kushner et al. 2007).
Morimoto et al. studied the transport pathway of fluorescently labeled hydrophilic dextran molecules (40 kDa) in hairless rat skin after low-frequency sonophoresis using confocal microscopy (Morimoto et al. 2005). Upon ultrasound exposure, dextran molecules penetrated into the skin, up to a depth of 20 μm. Several crack-like structures were observed in the sonicated skin that lied under the ultrasound transducer. Fluorescence of dextran in these structures as well as the hexagonally shaped units, presumably keratinocytes, was also seen. It was concluded that low-frequency sonophoresis increased the transdermal transport of hydrophilic solutes by causing a degree of structural alteration and then inducing convective solvent flow probably via both corneocytes and lipids of the stratum corneum as well as newly developed routes.
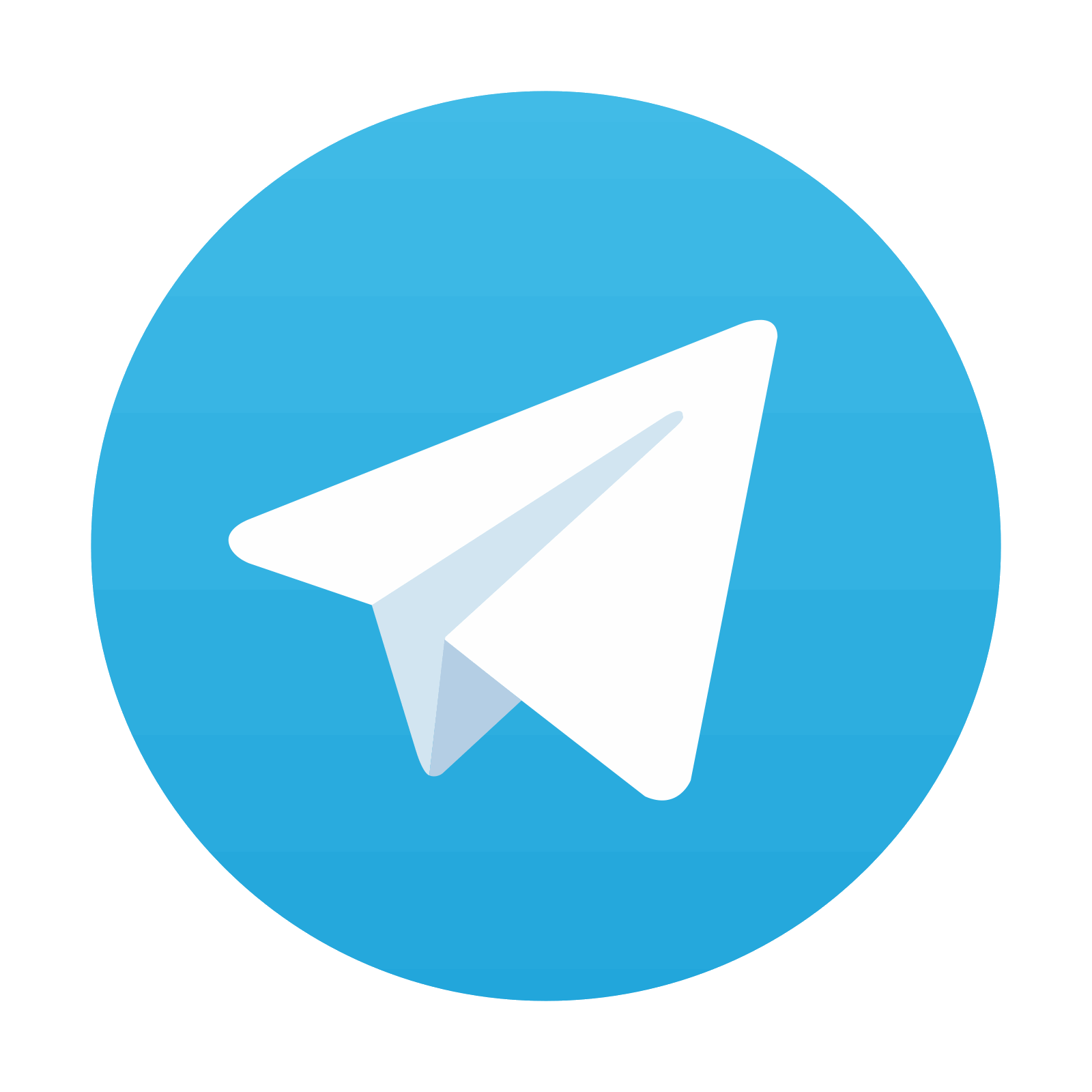
Stay updated, free articles. Join our Telegram channel
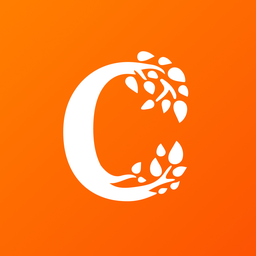
Full access? Get Clinical Tree
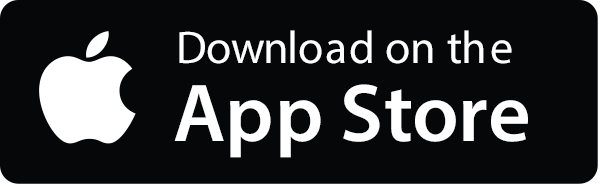
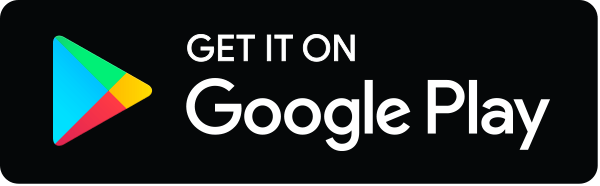