© Springer-Verlag Berlin Heidelberg 2017
Nina Dragicevic and Howard I. Maibach (eds.)Percutaneous Penetration Enhancers Physical Methods in Penetration Enhancementhttps://doi.org/10.1007/978-3-662-53273-7_2020. Evaluation of Microneedles in Human Subjects
Haripriya Kalluri1 , Seong-O Choi2 , Xin Dong Guo3 , Jeong Woo Lee4 , James Norman5 and Mark R. Prausnitz6
(1)
Research Scientist II, Georgia Institute of Technology, Atlanta, GA, USA
(2)
Department of Anatomy and Physiology, Nanotechnology Innovation Center of Kansas State, College of Veterinary Medicine, Kansas State University, Manhattan, KS, USA
(3)
College of Materials Science and Engineering, Beijing University of Chemical Technology, Beijing, China
(4)
Research Engineer II, Transdermal Drug Delivery Laboratory, School of Chemical and Biomolecular Engineering, Georgia Institute of Technology, Atlanta, GA, USA
(5)
Georgia Institute of Technology, Atlanta, GA, USA
(6)
Chemical and Biomolecular Engineering, Georgia Institute of Technology, Atlanta, GA, USA
Keywords
MicroneedleHuman studiesClinical trialTransdermal drug deliveryVaccineSkin needlingDisclaimer
James Norman’s work on this chapter was performed prior to his employment at FDA and does not represent the views and/or policies of the US Food and Drug Administration.
20.1 Introduction
Microneedles are micron-sized structures which create microscopic holes in the upper layers of the skin, thereby enhancing topical and transdermal delivery of therapeutic moieties. Since microneedles are minimally invasive, they usually do not stimulate nerve endings in the dermis, thereby offering a pain-free delivery system capable of administering small-molecule drugs, macromolecules, and vaccines. Based on their structural design, microneedles can be broadly categorized into solid and hollow microneedles, and they can be fabricated from a wide range of materials. As indicated by the literature on microneedles, a significant amount of data has been generated for a variety of microneedles from in vitro and in vivo animal studies pertaining to fabrication, characterization of microneedles, and drug/vaccine delivery (Kim et al. 2012; Pettis and Harvey 2012; van der Maaden et al. 2012; Donnelly et al. 2010; Sachdeva et al. 2011). However, certain aspects of microneedle technology such as pain associated with microneedles, patient and provider perceptions of this technology, safety concerns, and the efficacy of this technology in humans cannot be determined from animal studies. In recent years, several human studies and clinical trials have been performed to address these concerns and assess the efficacy of microneedles for drug and vaccine delivery in humans; these studies are discussed in this chapter.
20.2 Human Studies to Validate Microneedle Performance and Safety
20.2.1 Microneedle Insertion into the Skin
The most important function of microneedles is to overcome the barrier imposed by the skin’s outermost layer, stratum corneum, thereby facilitating the delivery of drugs into the body. This requires that microneedles puncture across the stratum corneum and into the skin. Many studies have shown that successful skin penetration by microneedles depends on several factors including microneedle geometry, material, applied force, and insertion strategy (Davis et al. 2004; Bal et al. 2008; Coulman et al. 2011; Haq et al. 2009). For example, microneedle geometry is an important factor that determines the force required for insertion without needle breakage. To achieve safe and reliable microneedle insertion, the force inducing mechanical failure of microneedles should be much higher than the insertion force of microneedles, which mainly depends on the tip radius, tip angle, and ratio between needle height and base width (Davis et al. 2004; Bal et al. 2008). Davis et al. demonstrated successful insertion of metal hollow microneedles in human subjects and indicated that the margin of safety (ratio between the fracture and insertion force) could be maximized with hollow metal microneedles having a small tip radius and a large wall thickness (Davis et al. 2004). Also, the work done by Bal et al. suggested that longer and sharp-tipped microneedles could make a deeper insertion (Bal et al. 2008), and similar results were found in other human studies as well (Coulman et al. 2011; Haq et al. 2009). In general, safe and reliable insertion can be achieved by microneedles bearing a small tip radius, acute tip angle, and high aspect ratio.
Skin deformation is also a barrier to successful microneedle insertion. Most incomplete insertions due to skin deformation occur when the aspect ratio of the microneedles is small or the microneedle length is short (Coulman et al. 2011). Typically, skin deformation during needle insertion could be overcome by increasing the needle length, applying higher force/speed during insertion, or utilizing especially designed applicators that provide constant force and minimize skin deformation (Bal et al. 2008; Coulman et al. 2011; Haq et al. 2009; Daddona et al. 2011). Since the degree of deformation depends on the location on the body, it may be desirable to consider different needle designs and application strategies depending on the needle insertion site.
The assessment of skin penetration by microneedles can be performed by several methods. Histological analysis has been widely used for in vitro and in vivo animal studies, but it is hard to be applied to human subjects because it requires skin excision. Also, it was reported that the dimensions of microchannels created by microneedles may be overestimated when visualized by histological techniques (Coulman et al. 2011).
Dye staining and electrical resistance measurements can indicate the disruption of the stratum corneum in vivo (Davis et al. 2004; Haq et al. 2009; Wermeling et al. 2008). In dye-staining studies, when the site porated with microneedles is stained with a dye such as methylene blue or gentian violet, only the disrupted areas of the stratum corneum are stained, thereby aiding in visualization of the created microchannels. Upon poration, the electrical resistance of the skin drops rapidly, and therefore these measurements can also be used to confirm successful barrier disruption. These methods, however, do not provide information about the three-dimensional penetration profile created by microneedles.
Transepidermal water loss (TEWL) measurements have also been used to evaluate the level of skin disruption (Bal et al. 2008; Haq et al. 2009). This method has been used in the cosmetics and dermatology industry to determine changes in skin barrier properties. Although TEWL measurement only provides the degree of skin damage in a qualitative manner, it is a useful tool to investigate the effect of microneedle geometries on skin disruption and resealing. Studies on human subjects have demonstrated that TEWL values dramatically increase upon microneedle insertion, and the highest TEWL values were obtained with longer needles and multiple treatments, indicating greater barrier disruption (Bal et al. 2008; Haq et al. 2009).
Recently, optical coherence tomography (OCT) has become an attractive way to investigate structural and biomechanical features of the skin. OCT is a noninvasive interferometric technique utilizing local optical backscatter for imaging and has been widely used in ophthalmology. Microchannels created by microneedles were successfully visualized using OCT, and the penetration depths ranged between 15 and 65 % of the full needle length depending on the needle geometry, needle arrangement and location, and insertion site (Coulman et al. 2011; Enfield et al. 2010), indicating that the biomechanical properties of a treatment site should be considered for effective skin disruption. It was also found that the width of the microchannel in the stratum corneum layer was approximately 50 times smaller than the width of the microneedle, thus implying that microneedle insertions are less invasive than predicted by histology results (Coulman et al. 2011).
20.2.2 Liquid Infusion Via Hollow Microneedles
Compared to drug delivery using solid microneedles, successful delivery of liquids via hollow microneedles is challenging because success relies not only on insertion of microneedles but also on issues associated with liquid infusion such as flow rate, volume, and leakage. Among various factors affecting infusion of liquids, management of leakage is of critical importance for successful liquid infusion using microneedles. To minimize leakage during infusion, microneedles should be long enough to ensure complete and secure insertion. However, the length of the needle should be short enough for minimizing pain associated with needle penetration.
Researchers have demonstrated successful liquid delivery into human subjects with microneedles varying in lengths from 0.5 to 3 mm (Laurent et al. 2007, 2010; van Damme et al. 2009; Gupta et al. 2011a, b, c). Laurent et al. reported that following infusion with a 1.5-mm-long, 30-gauge microneedles attached to a glass syringe which allows perpendicular insertion into the skin, more than 90 % of the injected liquid was deposited in dermal tissue, and a mean fluid leakage volume of 2–3 μL was observed (Laurent et al. 2007). Van Damme et al. demonstrated intradermal influenza vaccination using a silicon hollow microneedle array (450-μm tall, 1 x 4 array) (van Damme et al. 2009), and their immunogenicity data suggested that the leakage during injection was negligible, although they did not describe the amount of vaccine left on the skin surface after injection.
Another source of leakage is the skin itself since it provides significant resistance to fluid flow. The dermal layer has a limited capacity for accommodating fluid, which causes an increase of pressure in the dermis during injection. Therefore, it is important to understand the relationship between the accumulated pressure at the injection site and injection parameters such as flow rate and volume. Gupta et al. demonstrated that infusion pressure increased as more saline volume was delivered into the dermal layer, and the infusion pressure was independent of the insertion depth (Gupta et al. 2011a, b, c). They performed the experiments with glass hollow microneedles of three different lengths (0.5, 0.75, and 1 mm), and the results indicate that the leakage due to backflow could be managed by adjusting the flow rate, as long as the microneedles are securely inserted into the skin. Interestingly, there was a point where the infusion pressure was stabilized at low flow rate (0.1 mL/min). This suggests that there exists a steady state where the incoming flow rate is equal to the outgoing flow rate from the injection site into the body and, further, encourages the possibility of microneedle-assisted delivery of large liquid volumes over a period of time without increasing infusion pressure.
This study also demonstrated that infusion pressure could be lowered by partial microneedle retraction using a custom rotary device, which required an infusion pressure less than half of that required when using nonretracted microneedles. The pressure did not increase significantly for the retracted microneedles at larger infusion volumes (>0.6 mL). In addition, the delivery of a large volume of liquid via microneedles could be facilitated by the use of hyaluronidase, which is an enzyme that degrades hyaluronic acid in the extracellular matrix of the skin, thereby allowing the accommodation of additional fluid in the dermis. For small volumes (<0.3 mL), however, the pressure required to infuse into the skin was not significantly affected by microneedle length, flow rate, retraction, and the use of hyaluronidase, implying that a secure microneedle insertion is the most important factor for successful liquid infusion using hollow microneedles in human subjects.
20.2.3 Pain
One of the core advantages of microneedles over traditional injections is reduced pain and thereby increased patient compliance. Studies by various research groups have helped quantify this pain reduction and describe the key design factors affecting pain.
20.2.3.1 Solid Microneedles
The first studies on pain after microneedle applications were done with solid microneedles. Kaushik et al. compared 150-μm-long microneedles in a 20 × 20 array to a 2-mm, 26-gauge hypodermic needle (Kaushik et al. 2001). Twelve participants were blinded for manual needle insertions, and pain scores were recorded. Microneedles resulted in significantly less pain, with a median pain score of 0/100 compared to a score of 23/100 for hypodermic needle. Similar findings were observed by Haq et al., where two types of silicon microneedle arrays, each containing 36 needles measuring 180- or 280-μm long, were compared to a 25-gauge hypodermic needle in 13 participants (Haq et al. 2009). Both microneedle designs were less painful than the hypodermic needle. The 280-μm-long microneedles were considered less painful than the 180-μm-long microneedles, but the investigators intentionally applied more force when administering the shorter microneedles in order to ensure complete insertion.
Pain associated with various microneedle designs was investigated by Bal et al. in 18 participants (Bal et al. 2008). Microneedle patches with 16 microneedles, varying in length from 200 to 550 μm, were compared; no hypodermic needle was used for comparison. There was no significant difference in pain between the different microneedle designs, although the 550-μm-long microneedles had the highest median and maximum pain scores.
In a more detailed study, Gill et al. investigated various microneedle designs to determine which design factors affected pain (Gill et al. 2008). In this double-blinded study, 10 participants rated the pain of insertion associated with microneedles with lengths from 480 to 1450 μm, widths from 160 to 465 μm, thicknesses from 30 to 100 μm, tip angles from 20° to 90°, and array sizes from 1 to 50 microneedles. All microneedle designs tested were significantly less painful than a 26-gauge hypodermic needle, and microneedle length had the most significant effect on pain, followed by needle density, while width, thickness, and tip angle did not affect pain significantly over the range of parameters studied.
The primary takeaway message from these studies is that solid microneedle insertions universally result in less pain compared to hypodermic needles. Microneedle length is the primary factor affecting microneedle pain, but significant differences in pain among the various designs investigated did not appear until microneedle length approached 1 mm.
20.2.3.2 Hollow Microneedles
The use of hollow microneedles is mainly dependent on microneedle design and fluid flow parameters, which in turn affect pain associated with this mode of application. Gupta et al. investigated the effect of microneedle length, infusion volume, flow rate, needle retraction, and additional use of hyaluronidase on pain levels during infusion of saline in ten participants (Gupta et al. 2011a). Microneedle insertions were significantly less painful than hypodermic needle insertions regardless of length. Microneedle lengths from 500 to 1000 μm did not have a significant effect on infusion-related pain, except at a large infusion volume of 1.0 mL, where longer needles were reported to be more painful. Increasing the infusion volume also increased pain, while the flow rate did not affect pain significantly except at an infusion volume of 1.0 mL for which a sharp piercing pain was sometimes reported. Partial retraction of the microneedle prior to infusion was investigated to reduce infusion pressure by relieving tissue compaction caused by microneedle insertion. Partial retraction significantly reduced infusion pressure, but also significantly increased infusion pain, possibly related to increased fluid-mechanical micro-damage to the tissue due to increased fluid flow. Incorporation of hyaluronidase into the injection formulation significantly reduced pain for an infusion volume of 1.0 mL. Overall, when compared to intradermal infusion with a 26-gauge needle, microneedle infusions generally required greater pressure but caused less pain.
In another study with 645 participants, Laurent e t al. compared the pain associated with needle insertion and saline infusion for microneedle application (1.5-mm microneedle applied to the deltoid) versus the standard Mantoux technique for intradermal delivery (26-gauge, 3/8″ needle) (Laurent et al. 2007). Microneedle insertion was reported as pain-free for all participants; however, a faint burning-like sensation was sometimes reported during saline infusion. At least three additional studies have been reported that indicate hollow microneedle insertions as significantly less painful than hypodermic needle insertions (van Damme et al. 2009; Gupta et al. 2011a, c). For infusion of drugs through hollow microneedles, some studies have reported equal or greater VAS pain scores with the hollow microneedles compared to hypodermic needles (van Damme et al. 2009; Gupta et al. 2011a; Pettis et al. 2011a, b; Durando et al. 2012), while others reported significantly less infusion pain (Gupta et al. 2012; Prymula et al. 2012; Dhont et al. 2012), which probably depends on the degree of tissue deformation and micro-damage caused by different needle geometries and injection protocols.
Overall, insertion of solid and hollow microneedles can be done painlessly, but pain associated with infusion through hollow microneedles is variable and remains a potential source of discomfort for patients.
20.2.4 Safety
Microneedles have been shown to enhance the delivery of a wide range of molecules into the skin, including small molecules, peptides, vaccines, and plasmid DNA (Donnelly et al. 2010). However, in order to be clinically feasible as a drug delivery technique, it is important that microneedle treatment is both well tolerated by patients and safe with regard to any potential local skin irritation and systemic effects.
Skin irritation is defined as a nonimmunological local inflammatory reaction that is usually reversible and can lead to erythema and edema (Bal et al. 2008). Cytokines from epidermal cells play an important role in skin inflammatory processes, and keratinocytes, which comprise 95 % of the epidermal cells, produce a variety of cytokines in response to barrier disruption (Williams et al. 1996). Therefore, physical barrier disruption by microneedles may also potentially induce an inflammatory reaction. The degree of skin irritation can be assessed by various noninvasive biophysical techniques including visual inspection of skin color (Gill et al. 2008; Van Damme et al. 2010) and scoring methods, such as chromameter (Noh et al. 2010), laser Doppler imaging (Ansaldi et al. 2012; Corsini et al. 2000), reflectance spectroscopy (Noh et al. 2010), and visual scoring (Van Damme et al. 2010). There are several factors that may potentially affect the safety of microneedles, which include the type of microneedles (solid or hollow), microneedle dimensions (length, width, thickness, tip angle, number of needles in an array), and materials the microneedles are fabricated from (metal, silicon, glass, or biodegradable polymers).
In 2001, Kaushik et al. carried out the first human study with 150-μm-long silicon microneedles in 12 male and female healthy volunteers aged 18–40 years (Kaushik et al. 2001). The areas of the skin treated with microneedles were visually inspected post insertions, and neither redness nor swelling was observed in all cases, suggesting that the microneedle treatment did not cause significant tissue damage or irritation. On the other hand, hypodermic needle insertions always led to appearance of blood at the insertion site.
In another study, skin irritation associated with application of solid and hollow metal microneedle arrays of various lengths (200, 300, 400, and 550 μm) was investigated by Bal et al. in 18 healthy volunteers aged 21–30 years using chromameter and laser Doppler imaging methods (Bal et al. 2008). The hollow microneedles used in that study resulted in less skin irritation compared to the solid microneedles, and the shape and length of the microneedles affected the degree of irritation. A higher degree of erythema and blood flow was observed for 400-μm-long microneedles compared to 200-μm-long microneedles. However, in all cases, the irritation was minimal and lasted less than 2 h.
Gill et al. investigated the safety of longer solid metal microneedles with lengths of 480, 700, 960, and 1450 μm in human volunteers (Gill et al. 2008). Redness was observed for all microneedle insertions, but the erythema decreased in 2 h. A tiny droplet of blood was observed at the insertion site after some insertions with the 1450-μm-long microneedles, while the shorter microneedles did not result in any bleeding. There were no signs of edema after all microneedle insertions.
The clinical safety of even longer, hollow, metal microneedles (1–3-mm long) was also evaluated in 66 healthy adult volunteers with ages ranging from 18 to 45 years by a visual scoring method (Laurent et al. 2010). No serious adverse events were reported in the intramuscular injection and intradermal injection (microneedles; BD Soluvia™ Microinjection System) groups. Local pain at the injection sites was frequently reported in the intramuscular group but never in the intradermal group.
The safety of microneedles fabricated from metal (Bal et al. 2008; Gill et al. 2008), silicon (van Damme et al. 2009), and glass (Gupta et al. 2009, 2011a, 2012) has also been reported. For metal microneedles with heights lower than 960 μm, no or minimal local irritation was observed, which lasted less than 2 h (Bal et al. 2008; Gill et al. 2008). Van Damme et al. reported that for injection using hollow, silicon microneedles with a height of 450 μm, local reactions at the insertion site were frequent among recipients, but these reactions were mild and invariably transient (van Damme et al. 2009). Similar findings were observed for injection using hollow, glass microneedles (500–900-μm long) as well, where very mild erythema or edema was observed in the skin, but this irritation did not appear to be associated with an inflammatory response (Gupta et al. 2009, 2011a, 2012). In contrast, hypodermic needle insertions led to the presence of a drop of blood at the insertion site which was not observed for microneedle insertions.
Overall, compared with hypodermic needles, the use of microneedles is considered safe, owing to their small size and lack of significant damage to skin tissue and blood vessels, which means negligible pain, local irritation, or systemic reactions.
20.2.5 Skin Resealing
Following microneedle insertion, the time over which the created microchannels remain open is critical for optimal drug delivery. Ideally, the microchannels should remain open for the entire time when the patch or drug formulation is applied on the skin and should close soon thereafter to minimize risk of infection.
Skin resealing kinetics in humans was first extensively investigated by Gupta et al. using skin impedance measurements (Gupta et al. 2011b). Metal microneedles were inserted into the skin, and impedance measurements were monitored until they reached the baseline values indicating complete pore closure. Microchannels closed within 2 h of microneedle insertion when the treated site was left open to the environment. However, when the treated site was occluded, pore closure was delayed up to 3–40 h, depending on the microneedle geometry. Similar findings were observed by Wermeling et al. where, under occluded conditions, pore closure was reported around 30 h post insertion, as indicated by impedance measurements (Wermeling et al. 2008).
The effects of different microneedle lengths (500–1500 μm), dimensions (75- vs. 125-μm thickness; 200- vs. 500-μm width), and microneedle numbers (10 vs. 50) on pore closure were also investigated by Gupta et al. (2011b). The time for complete pore closure depended on the length of the microneedles, the number of microneedles, and the area of poration, which in turn characterizes the depth of poration and the degree of injury to the skin.
The lifetime of the pores and its effect on drug delivery was studied by Wermeling et al. (2008). In this study, six subjects were pretreated with 400 metal microneedles (620-μm long) followed by application of a naltrexone hydrochloride gel patch. Drug levels in the plasma indicated that pores were open for at least 48 h, and for 72 h in two subjects. Pharmacologically active drug levels were found in the plasma even at 72 h post patch placement. However, when skin resealing was investigated in another set of ten subjects who were treated with microneedles only, skin electrical measurements indicated that the pores remained open for up to 30 h. Therefore pores were open for at least 30 h, and the prolonged delivery of naltrexone up to 72 h could, for that reason, be attributed to a drug depot formation in the skin. This study shows the direct effect of pore lifetime on drug delivery.
Pore closure kinetics vary depending on the age of the subject. Kelchen et al. reported the micropore closure kinetics in 16 elderly subjects compared to control group. Data indicate longer time frames are required to restore skin barrier function, suggesting a longer window of opportunity for drug delivery in the elderly population (Kelchen et al. 2016).
In summary, following poration, pores close within a relatively short period of time when left open to the environment, thereby reducing risk of infection or other side effects. Pore closure can further be delayed by introducing occlusive conditions which may be beneficial for delivering drugs over extended periods of time. The time required for skin resealing is dependent on the dimensions, geometry, and number of microneedles applied; the age of the subject; and the degree of injury appears to determine the time required for complete skin resealing.
20.3 Human Studies of Drug and Vaccine Delivery
Drug delivery using microneedles can be achieved via different application modes. In the poke-and-patch approach, drug-free, solid microneedles are inserted into the skin creating microchannels, followed by application of a drug patch or drug formulation on the porated skin site, which then allows diffusion of the drug from the patch or formulation into the skin. In the second approach, solid microneedles can be coated with the drug formulation; once these needles are inserted into the skin, the interstitial fluid in the skin dissolves the coating, thereby depositing the drug directly in the skin. In the third approach, the drug can be encapsulated into a biodegradable matrix of dissolving microneedles. Upon insertion, these microneedles dissolve, depositing the drug in the skin. Finally, liquid formulations can be infused into the skin using hollow microneedles. The physicochemical properties of the drug moiety, duration of delivery (bolus or extended periods of time), dosage, and dosing regimen are some of the factors that may determine the best mode of microneedle application.
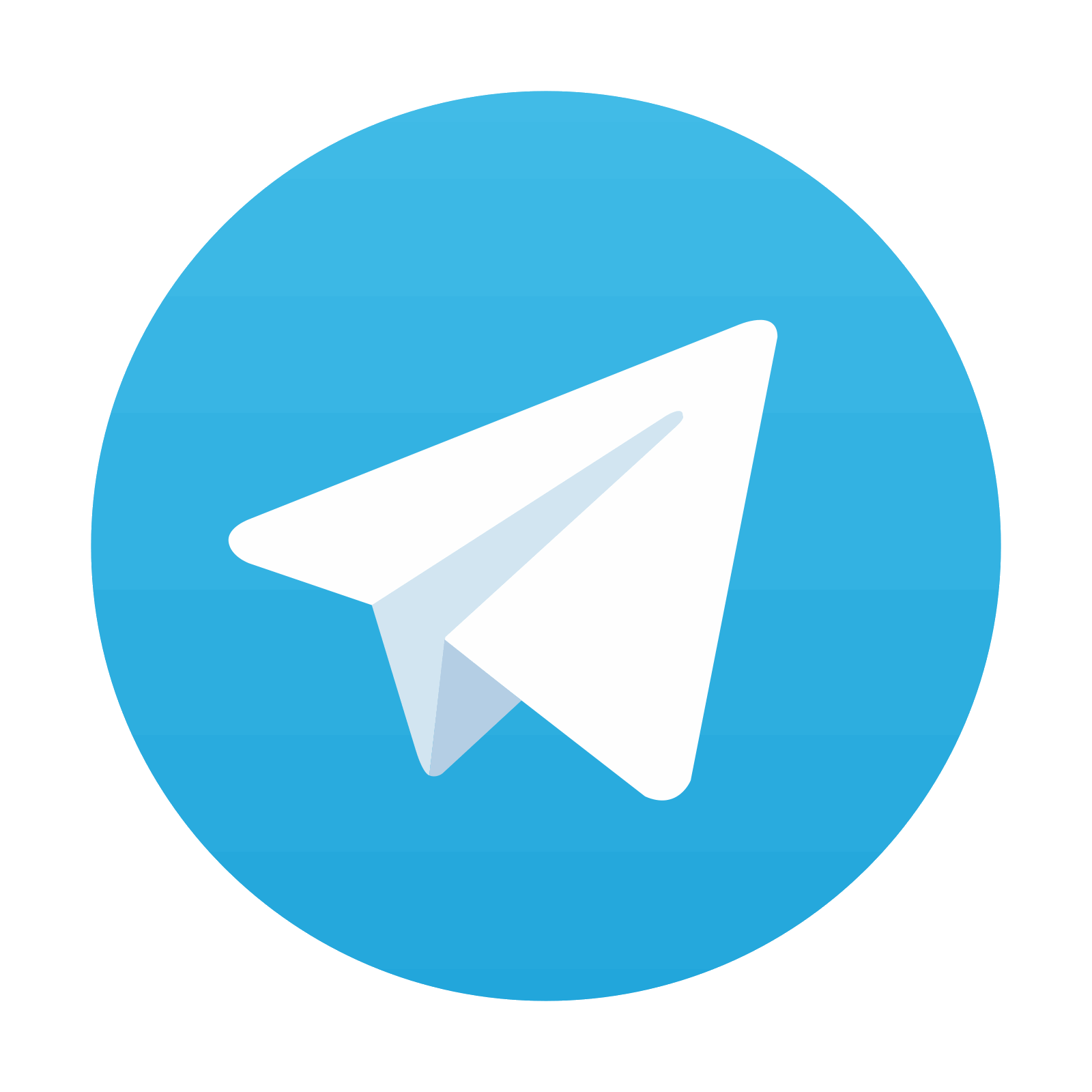
Stay updated, free articles. Join our Telegram channel
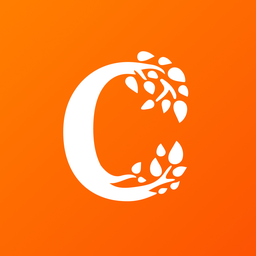
Full access? Get Clinical Tree
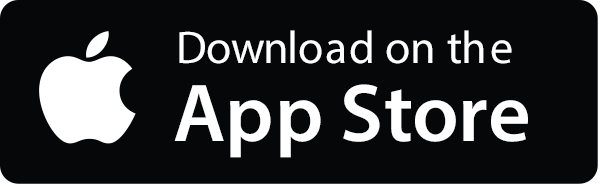
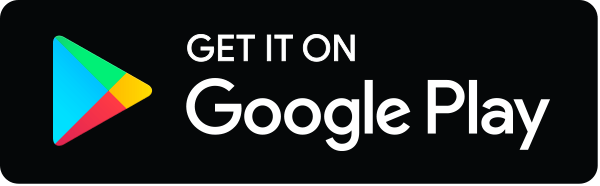