Enhancement technology
Devices/products
Company
Microneedles
Macroflux® technology
Zosano Pharma (USA)
sMTS and hMTS technology
3 M (USA)
DermaRoller®
DermaRoller (USA)
MicroCorTM
Corium (USA)
BD SoluviaTM
Becton Dickinson (USA)
Soluble microneedles
Elegaphy (Japan)
MicronJet needles (hollow)
MicroPyramid platform
Nanopass (Israel)
Iontophoresis
IsisIQTM
Isis Biopolymer (USA)
LidoSite®
Vyteris (USA)
Lidoderm®, Iontopatch®
Teikoku Pharma (USA)
Empi Action Patch™
Empi (USA)
IDDS
Dharma Therapeutics (USA)
Transderm® Ionto System
Mattioli Engineering (USA, Germany, Italy)
Trivarion®, ActivaPatch®
ActivaTek™ (USA)
Sonophoresis
SonoPrep® (withdrawn)
Echo Therapeutics (USA)
U-StripTM and U-WandTM
Transdermal Specialties (USA)
Jet injector
GentleJet
Activa (Canada)
GentleJet, Medi-Jector VISION®
Antares (USA)
Biojector®, ZetaJet, cool.clickTM
BioJect (USA)
SQ-PEN
The Medical House PLC (UK)
Laser ablation
P.L.E.A.S.E.®
Pantec Biosolutions AG (Liechtenstein)
Epiture Easytouch TM
Norwood Abbey (Australia)
Thermal ablation
Passport® Patch
Altea Therapeutics (USA)
Radio-frequency ablation
ViaDermTM
TransPharma Medical (Israel)
27.2 General Considerations for Transdermal Delivery of Peptides and Proteins
Protein-based biopharmaceuticals include hormones, enzymes, cytokines, antibodies, and vaccines (Table 27.2). Understanding the protein/peptide structure including protein size, shape, isoelectric point (pI), amino acid sequence, and three-dimensional structures is essential for the choice of transdermal enhancement techniques and design of formulations. Most therapeutic proteins are globular proteins that are well compacted. However, their size is not small enough to pass through the tight intercellular junctions in the stratum corneum. Therefore, additional driving force or pathways are required to support their transdermal delivery. The pI of peptides is also an important consideration especially for iontophoresis-assisted transdermal delivery of peptides. This will be discussed in more detail in the iontophoresis section. In addition to the physicochemical properties of proteins, formulation factors also play an important role in transdermal delivery of proteins. The pH, ionic strength of buffers used in the formulation, protein concentration, formulation viscosity, and the presence of surfactants influence the stability of protein drugs and in turn influence their transdermal delivery. An ideal formulation should avoid protein aggregation and degradation during shelf life and maintain bioactivity throughout the transport of proteins across the skin. Another important factor affecting the efficacy of transdermal protein delivery is dermal metabolism. In general, the skin has much lower enzyme activity as compared to mucosa and gastrointestinal tract. However, topically applied peptides and proteins are still subjected to considerable enzymatic degradation compromising their transdermal delivery. Such enzyme-sensitive peptides and proteins include thyrotropin-releasing hormone, vasopressin, luteinizing hormone-releasing hormone, and bovine serum albumin (BSA) (Huang and Wu 1996; Banga et al. 1995; Bachhav and Kalia 2009; Pikal and Shah 1990; Choi et al. 1990). Addition of protease inhibitors such as aprotinin to the formulation may help address the issue of proteolytic degradation of peptides and proteins during their transport across the skin.
Table 27.2
Therapeutic peptides and proteins investigated for transdermal route
Peptide/protein | MW (Da) | pI | Current route | Indications |
---|---|---|---|---|
Hormones | ||||
Thyrotropin-releasing hormone (TRH) | 362 | – | iv. | Adjunctive agent in diagnosis of thyroid function |
Vasopressin and analogues | 1050 | 10.9 | im. | Enuresis, polyuria, diabetes insipidus, etc. |
Luteinizing hormone-releasing hormone (LHRH or GnRH) | 1182 | 9.6 | iv. | Infertility |
Leuprolide | 1209 | 9.2 | sc. im. | Prostate cancer, endometriosis, uterine fibroids |
Salmon calcitonin | 3431 | 10.4 | im. nasal | Postmenopausal osteoporosis Paget’s disease |
Insulin | 6000 | 5.4 | iv. sc. oral | Diabetes |
Parathyroid hormone | 9420 | 5 | sc. | Osteoporosis |
Follicle-stimulating hormone (FSH) | 34,000 | 7.5 | im. | Infertility |
Human growth hormone (hGH) | 22,000 | 5.3 | sc. | hGH deficiency, Turner syndrome |
Growth factors and cytokines | ||||
Epidermal growth factor | 6000 | 4.6 | Topical | Wound healing |
Human basic fibroblast growth factor | 17,400 | 9.6 | Topical | Wound healing |
Interferon-α-2b | 19,000 | 6 | iv. | Chronic hepatitis C, leukemia, acquired immunodeficiency syndrome-related Kaposi’s sarcoma |
Interferon-β-1a | 22,500 | 8.9 | iv. | Multiple sclerosis, condyloma acuminatum |
Erythropoietin | 18,400 | 8.6 | iv. sc. | Anemia |
Antibodies | ||||
Immunoglobulin G | 150,000 | 6.1–8.5 | iv. | Immunomodulation |
27.3 Physical Enhancement Techniques Assisting Transdermal Delivery of Peptides and Proteins
27.3.1 Microneedles
Over the past decade, microneedles have been developed as a minimally invasive and painless technology for transdermal drug delivery. Microneedles are tiny needles used to create micron-sized pores in the skin. These pores result in disruption of the stratum corneum layer bypassing the rate-limiting barrier for transdermal permeation and enabling the transport of macromolecules across the skin. Microneedles vary in length and shape but typically create microchannels that penetrate through the stratum corneum and a portion of the epidermis. Since these needles do not reach the dermis layer of the skin containing nerves and blood vessels, microporation is a painless enhancement technique for transdermal delivery. The pores created are transient as the stratum corneum naturally recovers through desquamation. Thus, this technique is painless and convenient and offers a highly promising solution for transdermal delivery of a variety of macromolecules that are not effectively delivered by passive transdermal delivery.
In 1998 Henry et al. published the first study on the use of microneedles for transdermal drug delivery and reported increased permeability of the human skin for model hydrophilic drug calcein (Henry et al. 1998). Since then, there has been increasing interest in this technology, and it has been used to deliver a wide range of drug molecules including hydrophilic compounds and macromolecules into and across the skin (Prausnitz and Langer 2008; Gupta et al. 2011; Zhang et al. 2010; Duan et al. 2011; Banga 2009). The reason behind this increased interest in microneedle-assisted transdermal drug delivery can be attributed to both potential benefits of this technology for patients and technological advances in microfabrication technology exploring range of materials for a variety of sizes and shapes of needles (Saurer et al. 2010; Chu et al. 2010; Lee and Jung 2012). Microneedles may be as solid or hollow in design. They can be fabricated into a variety of geometries and dimensions and can be made from several materials including silicon, metals, polymers, and sugars. Depending on microneedle design, there are four types of approaches to apply microneedles, “poke and patch” or “coat and poke” for solid microneedles, “poke and dissolve” for dissolving microneedles, as well as “poke and flow” approach for hollow microneedles (van der Maaden et al. 2012).
We briefly summarized some of the recent work on the use of microneedles for transdermal delivery of peptides and protein below. Tas et al. have reported development of a transdermal patch containing microneedles coated with peptide, salmon calcitonin (sCT) as an alternative to conventional subcutaneous and nasal delivery route using hairless rat model (Tas et al. 2012). They reported that these microneedles delivered similar amounts of sCT compared to subcutaneous administration and significantly higher amounts of sCT in comparison to nasal administration. Ito et al. have employed insulin-loaded dissolving microneedles for transdermal administration of insulin (Ito et al. 2012). The hypoglycemic effect in rat after delivery of insulin through microneedles was compared with subcutaneous injection. They reported relative pharmacological availability of approximately 98 % for insulin using two-layered dissolving microneedles. Similarly, Martanto et al. reported decrease in blood glucose level of up to 80 % in diabetic rats following transdermal administration of insulin through hollow and solid metal microneedles (Martanto et al. 2004; Davis et al. 2005). Similarly, other researchers have reported successful microneedle-mediated delivery of protein and peptides including parathyroid hormone, desmopressin, insulin, human growth hormone, human immunoglobulin (IgG), and erythropoietin through the skin (Ito et al. 2012; Cormier et al. 2004; Li et al. 2009; Daddona et al. 2011; Kumar and Banga 2012; Peters et al. 2012). Several other companies including 3 M and Becton Dickinson are currently developing this technology for transdermal delivery of a number of macromolecules (see Table 27.1).
The delivery of protein and peptides with microneedles can be affected by several parameters including length of microneedles, number of microneedles in a unit area, drug formulation, and basic design/geometry of microneedles. Microneedle length is an important parameter that controls the depth of microchannels and subsequent drug delivery through these microchannels. Typically, microneedles of length ranging from 50 to 900 μm have been reported in literature (Banga 2011). An applicator or an insertion device may be required to insert shorter microneedles (<200 μm), while longer needles can be applied manually. The speed and time of application are also contributing factors that govern the total force applied on to the needles to porate the skin. Needle density (number of microneedles in unit area) also affects the efficacy of application. If there are many needles in small area and needle-to-needle spacing is too small, the pressure applied on each microneedle is compromised creating a bed-of-nails effect and resulting in insufficient force to penetrate the skin.
Although insertion of microneedles is generally painless, optimization of needle length and density is required to avoid possible injury or pain. There are published studies investigating pain associated with microneedle application (Gill and Prausnitz 2007; Bal et al. 2008). Bal et al. have shown that application of 550 μm length solid and assembled microneedles was effective in breaching the skin barrier in human volunteers without causing any pain and had minimal skin irritation issues (Bal et al. 2008). Kaushik et al. carried out a small trial to determine if microneedles are perceived as painless by human subjects. An array of 150 μm long microneedles (400 needles in an area of 3 cm × 3 cm) was inserted into the skin of subjects and compared to pressing a flat surface against the skin (negative control) and inserting a 26-gauge hypodermic needle into the skin surface (positive control). Subjects were unable to distinguish between the painless sensation of the flat surface and application of microneedles (Kaushik et al. 2001).
Following microporation, the skin starts to heal and regain its barrier properties, but time required for complete closure of micropores is a critical factor as open pores may cause issues such as irritation and infection. Kalluri and Banga have studied pore closure in hairless rats with soluble microneedles (Kalluri and Banga 2011a, b). They reported that the skin begins reestablishing its barrier functions in 3–4 h after microneedle treatment, but complete closure of pore takes up to 15 h. They also observed that occlusion with a plastic film or any solution delayed pore closure for up to 72 h. Thus, the microchannels allow drug delivery, while drug formulation is applied and pores start to close at faster rate once solution is removed. Haq et al. have studied the microchannel repair and resealing in human subjects with microneedles array (Haq et al. 2009). They also reported evident sign of microchannel repair and complete resealing within 8–24 h after application. Considering the advantages and safety of this technology, microneedles have important implication in transdermal delivery of peptides and proteins.
27.3.2 Iontophoresis
Iontophoresis is the use of mild current (generally less than 0.5 mA/cm2) to propel drug molecules into the skin. This technique provides good potential for transdermal delivery of various peptides and small proteins. Iontophoresis enhances drug permeation via two mechanisms – electromigration and electroosmosis. Electromigration is the repulsion of charged molecules by the electrode with the same charge. It is the major mechanism for iontophoretic delivery of protein, which is reported to account for over 70 % of total permeation of peptides and proteins (Abla et al. 2005; Cazares-Delgadillo et al. 2007). Electroosmosis refers to a bulk flow of water from anode to cathode under the influence of electric current. Since the skin is negatively charged, the movement of positively charged ions across the skin is favored. These ions such as sodium carry water of hydration along with them as they are transported across the skin under the influence of current. Hydrophilic molecules of therapeutic interest may be solvated by this water of hydration and transported into and across the skin.
Iontophoresis-mediated transdermal delivery of peptides and proteins is dependent on the physicochemical properties of these biomolecules. Iontophoresis can assist transdermal delivery of proteins ranging up to ~15 kDa (Kalluri and Banga 2011b). However, additional enhancement techniques may be required to deliver larger proteins or monoclonal antibodies across the skin using this enhancement technique. The isoelectric point or pI of peptides is another important consideration for iontophoretic transdermal delivery. Iontophoresis is challenging if the pI of protein is in the range of 4–7.4 (Lee et al. 1995). This is because the pH of the skin ranges from 4 at the surface of the skin to 7.4 in the dermis. Therefore, proteins with pI of 4–7.4 tend to lose charge as they move across the skin (at pI = pH). Proteins with pI lower than 4 and higher than 7.4 would remain charged throughout their transport across the skin and are better candidates for iontophoretic delivery. Vasopressin having pI of 10.9 (Nair and Panchagnula 2003; Schuetz et al. 2005) and salmon calcitonin having pI of 10.4 (Chaturvedula et al. 2005) are hence good candidates for iontophoretic transdermal delivery.
In addition to physicochemical properties, formulation parameters also play an important role in optimizing iontophoretic transdermal delivery. If electromigration is predominant, the iontophoretic flux is proportional to the current applied as per Faraday’s law (Phipps et al. 1989; Mudry et al. 2006):

where ti and zi are the transport number and valence of ion i, respectively, I is the current applied, and F is Faraday’s constant. Transport number is the fraction of the total current transported by a specific ion. Therefore the protein concentration and presence of other ions in the formulation will affect iontophoretic delivery. Increase in protein concentration can improve iontophoretic delivery, but high protein concentration may also result in aggregation. Pillai et al. studied the effect of insulin concentration on its iontophoretic transdermal delivery. Optimal permeation of insulin was obtained at the concentration of 75 IU/ml. Further increase in insulin concentration compromised transdermal delivery (Pillai et al. 2003). Another important factor influencing protein delivery is ionic strength of the formulation. Co-ions and counterions will compete with peptides and proteins for transport due to relatively low mobility of these macromolecules. In general, a decrease of ionic strength is favored to reduce this competitive effect. However, certain levels of salts are required to maintain the formulation conductivity and protein solubility.

(27.1)
Iontophoresis parameters also influence transdermal delivery. Most studies use direct current (DC) iontophoresis although it has some limitations such as limited application time, increase in impedance, and change of skin biology. Pulsed DC and alternating current (AC) iontophoresis were also examined. These conditions were shown to avoid skin polarization, prolong treatment duration, and reduce discomforts of patients. Raiman et al. found that 75 % pulsed DC resulted in significantly higher delivery of LHRH and nafarelin as compared to DC iontophoresis, whereas AC iontophoresis showed lowest permeation (Raiman et al. 2004). On the contrary, Lvovich et al. reported AC iontophoresis resulted in higher permeation of insulin as compared to DC iontophoresis (Lvovich et al. 2010). Thus, the enhancement following different types of iontophoresis may vary case by case.
Iontophoresis is used to assist delivery of leuprolide at different pH (Kochhar and Imanidis 2004). The optimal permeation was obtained at pH 7.2. Due to the competition of buffer salts, only 1 % of the current actually assisted leuprolide delivery even after high molecular weight buffer system was used. However, 0.8 μg/cm2/h of leuprolide can be delivered. Therapeutic dose can be reached using a permeation area of 10 cm2. A more recent study investigated the iontophoretic delivery of human basic fibroblast growth factor (hbFGF, 17.4 kDa) (Dubey et al. 2011). The protein remains intact during iontophoresis. Following 8 h iontophoresis at the current of 0.5 mA/cm2, 17.64 μg/cm2 of hbFGF was able to permeate across dermatomed porcine ear skin, whereas 77.74 μg/cm2 was deposited in the skin. Dubey and Kalia compared the iontophoretic delivery of ribonuclease A and ribonuclease T1 which have similar property and biological function with opposite surface charge (Dubey and Kalia 2010, 2011). Ribonuclease A has the pI of 8.64 and mass/charge ratio of 5.26. Anodal iontophoresis was performed at the current intensity of 0.5 mA/cm2 over 8 h using dermatomed porcine ear skin. The cumulative permeation and skin deposition of ribonuclease A were 252.19 and 206.40 μg/cm2, respectively. Ribonuclease T1 with the pI of 4.67 and mass/charge ratio of 9.03 was also delivered using cathode iontophoresis. The permeation in and across the skin was significantly reduced as compared to ribonuclease A due to the effect of electroosmosis.
Several iontophoresis devices are available on the market. LidoSite® – lidocaine HCl/epinephrine topical iontophoretic patch (Vyteris Inc., Fair Lawn, NJ, USA) – is used for providing analgesia prior to superficial dermatological procedures. Iontopatch® is a single-use wearable electronic disposable drug delivery (WEDD®) developed by Travanti Pharma (St. Paul, Minnesota), which is a subsidiary of Teikoku Pharma USA (San Jose, California). Recently, Smart Patch (Vyteris, Inc., USA) has been developed to deliver human gonadotropin-releasing hormone (GnRH) for infertility. Phase II clinical trials were completed in 2010 and have showed encouraging results.
27.3.3 Electroporation
Electroporation involves application of high-voltage electric pulses (100–1000 V) for a very short duration (1–100 ms) to create transient pores in the lipid bilayers of the stratum corneum and permeabilize the skin (Prausnitz et al. 1993). This technique has been extensively used in the past to deliver genetic material into cells. Recently electroporation has found its role in transdermal delivery.
Both electroporation and iontophoresis thus utilize the electric current to deliver biomolecules across the skin, but they differ markedly in their mode of action. Iontophoresis relies on the charge of the biomolecule to repel it into and across the skin layers, while electroporation uses high voltage to reduce skin resistance by increasing its permeability to allow the delivery of biomolecules. The key electrical parameters to be considered during electroporation are voltage intensity, pulse duration, and number of pulse. Properties of protein and peptides such as molecular weight, charge, and lipophilicity also influence their electroporation-assisted transport across the skin (Escobar-Chavez et al. 2009; Singh et al. 2012).
Studies have been reported utilizing electroporation for transdermal protein delivery. Zhao et al. have utilized electroporation as needle-free method to deliver peptide vaccine in a mouse model (Zhao et al. 2006). They reported the efficacy of this method was comparable to that of intradermal injection. Combination of this technique with other enhancement techniques such as iontophoresis has been reported to be more effective than either enhancement method alone. Medi and Singh have studied the synergistic effect of iontophoresis in combination with electroporation for transdermal delivery of human parathyroid hormone (hPTH). They reported several-fold increase in flux of hPTH across porcine skin by application of electroporation pulse followed by iontophoresis (Medi and Singh 2003). Similarly Chang et al. have reported quick and enhanced flux of salmon calcitonin through human epidermis by pulsing with 15 pulses of 500 V followed by 4 h of iontophoresis at an intensity of 0.5 mA/cm2 (Chang et al. 2000).
Although electroporation has the potential to be a promising technique for protein and peptide delivery across the skin, its use is limited. Pore formation in the skin due to electroporation may be reversible or irreversible. Higher-voltage electric pulses may result in formation of more aqueous channels in the skin which are of comparatively larger dimensions and may result in irreversible skin damage. In case of reversible pore formation, time required for the skin to recover from permeabilized state may vary significantly. The lack of portable/handheld devices for electroporation also limits the application of this technique for transdermal delivery of peptides and proteins.
27.3.4 Sonophoresis
Sonophoresis, or phonophoresis, uses ultrasound to propel the drug into/through the skin. Ultrasound is typically conducted from the source to the skin via a coupling medium, which is typically an aqueous formulation and may or may not contain a drug. Thus, the skin may be initially permeabilized by sonophoresis followed by passive drug delivery, or sonophoresis may be applied using the drug formulation as the coupling medium. Transdermal delivery following sonophoresis is known to be inversely proportional to the ultrasound frequency, and hence low-frequency ultrasound (20–100 kHz) was found to be most effective to deliver drug molecules across the skin (Tezel et al. 2001). The exact mechanism behind sonophoretic enhancement of transdermal delivery has not been elucidated. However, low-frequency ultrasound is thought to enhance drug delivery via the microcavitation mechanism. Ultrasound in the low-frequency range results in cavitation or formation of high-energy air bubbles in the coupling medium. These bubbles then impinge on the skin resulting in disruption of stratum corneum. It has been reported that low-frequency sonophoresis can result in creation of localized transport regions in the epidermis which are believed to be aqueous in nature (Weimann and Wu 2002; Tezel et al. 2004). Thus, sonophoresis provides good potential on transdermal protein delivery. Since sonophoresis is an energy-assisted technology, its enhancement effect is affected by the intensity, duty cycle, and application time as expressed in Eq. 27.2:
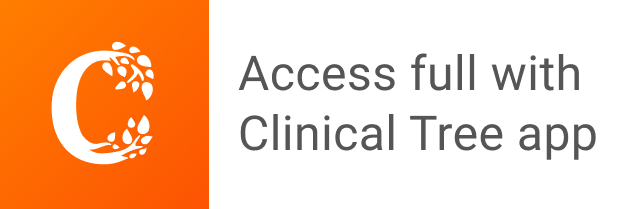