Fig. 17.1
Optical coherence topography (OCT) images showing microporation of human skin by using polymeric MN array (with height 600 μm, width at base 300 μm, spacing 300 μm) in (a) 2D and (b) 3D. Scale bar is 300 μm in each case (Reprinted with permission from Elsevier, Donnelly et al. 2010)
17.2 Mechanism of Creating Micropores by Different Microporation Devices
Microporation devices are considered to be noninvasive or minimally invasive in nature and therefore its application is painless. Drugs delivered using these minimally invasive or noninvasive microporation devices are generally absorbed into the body as quickly as the drugs administered by conventional subcutaneous needle injection (Brearley et al. 2007; Harris et al. 2006). Different microporation techniques work on different principles, but all techniques have one common goal, which is to disturb the principle barrier of the skin, i.e. SC by creating micropores (Fig. 17.2). Once created these micropores or pathways are orders of magnitude bigger than molecular dimension and therefore should readily permit transport of macromolecules as well as possibly supramolecular complexes and microparticles (Bouwstra et al. 1994). For drug delivery applications, microporation of the skin by different devices can be divided into two categories, i.e. mechanical method or active method. Mechanical method-dependent microporation technique involves the use of the MN-based devices. In contrast, thermal, radio frequency, ultrasound/phonophoresis, high-pressure jet and electroporation are based on active microporation techniques. The following sections detail the mechanism of microporation by different devices; however, readers are requested to refer relevant chapters in this book for more detailed studies on enhanced drug delivery achieved by using these devices.


Fig. 17.2
(a) Image showing penetration of microjets into the human skin in vitro. It also shows the intact structure of corneocytes around the injection site. The image was taken 15–30 min postinjection. Scale bar is 200 μm (Arora et al. 2007). (b) Scanning electron microscopy of radio frequency (RF)-microchannels in heat-separated epidermal membrane following two applications of ViaDerm™, high magnification showing dimensions of microchannels; scale bar is 100 μm (Birchall et al. 2006). (c) Image of micropore arrays on pig cadaver skin after 30-min delivery of sulforhodamine to thermally ablated skin. Scale bar is 1000 μm (Lee et al. 2011). (d) Scanning electron microscopy image of a single pore following laser microporation in mouse skin, generated by delivery of eight pulses at 0.76 J/cm2 per pulse. Scale bar is 50 μm (Reprinted with permission from Elsevier, Weiss et al. 2012)
17.2.1 Active Microporation Techniques
Active microporation of the skin can be achieved by using external source of energy. Therefore, these methods require much more complicated devices than the mechanical-based microporation devices, which need physical application to create micropores or microchannels, as shown in Fig. 17.2.
17.2.1.1 High-Pressure Gas or Liquid Microporation
Invented in the 1940s, the high-pressure jet injectors are perhaps the oldest microporation device intended to eliminate the directly mechanical piercing of the skin by hypodermic needles and/or syringes. Jet injections employ a high-speed jet to puncture the skin and deliver drugs without the use of a needle (Baxter and Mitragotri 2005). Injectors can be broadly classified as either multi-use nozzle jet injectors (MUNJIs) or disposable-cartridge jet injectors (DCJIs) (Baxter and Mitragotri 2005; Mitragotri 2006). These devices basically consist of a power source, usually a compressed gas and spring or piezoelectric actuator which upon actuation pushes a piston, which in turn impacts on a drug-loaded compartment, causing a surge in pressure and release of the drug-containing vehicle through a nozzle as a jet at a speed of between 100 and 200 ms−1 (Mitragotri 2006). The jet creates micropores upon impinging on the skin and delivers the drug at a depth dependent, either directly into muscles or subcutaneous or intradermal layers, on the characteristics of the jet, namely, orifice diameter, jet exit velocity and distance travelled. Micropore diameter is comparable to the jet diameter, which increases with increase in the distance travelled. The skin erosion and fracture are the root causes of micropore formation, as shown in Fig. 17.2a (Baxter and Mitragotri 2005). Several patents are in place for this method of drug delivery, in addition to the multitude of delivery devices already available, some of which are listed below under their trademark names: PenJet®, J-Tip®, Cross-Ject®, PowderJect®, MediJect®, Injex 30®, MHI-500®, LectraJet® and Impla-Ject®.
Literature review indicates enhancement of numerous drug molecules has been achieved by utilising this technique. However, in addition to drug delivery, jet injectors have also been proposed for the delivery of anti-ageing cosmetic products. For example, have proposed needle-free injector kits and quantities of dermal filling material for use in soft tissue augmentation. More specifically, the needle-free injectors allow injection of viscous materials, such as collagen, hyaluronic acid and other polymers that are useful as dermal fillers to fill undesired lines, wrinkles and folds.
17.2.1.2 Radio Frequency Microporation
Percutaneous penetration can also be facilitated by ablation of the outer layers of the skin by using alternating electrical current at radio frequency (RF) of 100–500 kHz. The passage of this current through cells in the upper skin strata, via an array of microelectrodes placed on the skin, propagates ionic vibrations through skin cells resulting in local heating, liquid evaporation and removal of cells. As a result, transient aqueous microchannels are created across SC and epidermis, called RF-microchannels (Fig. 17.2b), which enable or augment effective movement of water-soluble substances through the skin. Compared to other electrically assisted drug delivery techniques, such as electroporation and iontophoresis, microchannels formed using RF ablation are relatively large which enables transport of high-molecular weight compounds without the need for ionising or polarising the molecules. Furthermore, the formed microchannels do not reach underlying nerve endings and blood vessels; therefore, skin trauma and neural stimulations are minimised like with other microporation techniques discussed here (Sintov et al. 2003; Levin et al. 2005). Additionally, combination of RF-microchannel generation in conjunction with iontophoresis has also been studied (Levin et al. 2007a, b). For example, pretreatment of the human skin in vivo using ViaDermTM, a RF-microporation-based device, followed by iontophoretic patch application was reported to facilitate insulin delivery by a factor of 2.5 compared to ViaDermTM treatment alone (Levin et al. 2007a, b).
17.2.1.3 Thermal Microporation
It has been well documented that the flux of drugs through the skin is temperature sensitive and this factor has been utilised in transdermal and other types of delivery systems in recent times. This began with patented transdermal patch devices, which used heat to formulate the patch, rather than to increase the flux of drug across the skin (Konno et al. 1987; Kuratomi and Miyauchi 1988; Stewart 1989). In contrast, microporation of biological membrane was used to enhance drug delivery. Thermal microporation of skin involves application of rapid and controlled pulses of thermal energy by means of tiny resistive elements to a defined site on the skin surface to create micropores. The thermal energy will be passed through the array of tiny elements for few milliseconds, which causes flash vaporisation of SC cells in an area about the width of a human hair to create micropores (Fig. 17.2c) (Banga 2006).
Devices that directly used heat to increase the flux of drug across the skin became more prevalent in the 1990s. In the last 20 years, many new devices and methods have been established that utilise the microporation effects that are achieved when thermal energy is focussed on the skin and other biological membranes. In recent years more advanced devices have been developed, with some reaching full clinical trial and mass-scale production such as Altea PassportTM system. Furthermore, a combination of thermal microporation with other techniques has demonstrated much-improved enhancement than use of thermal microporation alone.
17.2.1.4 Laser Microporation
Ablation of the skin can also be achieved by using a laser emitted at a defined wavelength, which is directly absorbed by the tissue to form micropores, where irradiation of laser energy causes instant tissue vaporisation due to flash evaporation of water within in the irritated area following microexplosion that results in tissue ablation (Fig. 17.2d) (Nelson et al. 1991). It is this rapid energy loss from the ablated site, which protects the surrounding tissue from heat-induced damage. The two optimal wavelengths at which skin ablation can be achieved are short-wavelength ultraviolet and mid-infrared, which is absorbed by tissue proteins and tissue water, respectively. The amount of SC removal can be efficiently controlled by controlling the level of energy imparted on the skin, especially when applied at lower energy levels (Nelson et al. 1991).
17.2.1.5 Ultrasound (Phonophoresis, Sonophoresis) Microporation
Ultrasound is defined as sound with frequency ranging from 0.02 to 10.0 MHz and an intensity range of 0.0–3.0 W/cm2 (Mitragotri et al. 2000). Sonophoresis, also known as phonophoresis, describes the effects of ultrasound on the movement of drugs through intact skin and into soft tissues (Ng and Lui 2002). Ultrasound-enhanced drug delivery has several important advantages in that it is noninvasive, can be carefully controlled and can penetrate to desired depths into the body. The early use of ultrasound as a physical enhancer in transdermal drug delivery is developed nearly 50 years ago, a method referred to as sonophoresis or phonophoresis (Ng and Lui 2002). Exposure of a biological membrane to ultrasound causes sonoporation, which is the temporary, non-destructive perforation of the cell membrane. This transient state enhances permeability of therapeutic agents into cells and tissues (Harvey et al. 2002). However, the preceding is not intended to digress from the major principal of the review; however, before we emphasise the ultrasound-patented drug delivery systems, it is important to situate these systems in their contextual background.
Ultrasound is produced by a transducer composed of a piezoelectric crystal, which defines the frequency of emitted waves and converts electric energy into mechanical energy in the form of oscillations, generating acoustic waves. During the propagation of these acoustic waves through a given medium, a wave is partially scattered and absorbed by the medium, resulting in attenuation of the emitted wave with the lost energy being converted into heat ultrasound which can be emitted either continuously (continuous mode) or in a sequential mode (pulsed mode) (Machet and Boucaud 2002). The mechanism of ultrasound effects on the skin in drug delivery is not clearly understood; however, various different mechanisms were proposed (Lavon and Kost 2004; Tachibana and Tachibana 1999; Joshi and Raje 2002), as follows:
- (i)
Cavitation: Ultrasound generates gaseous cavities in a medium, and their subsequent collapse causes release of shock wave, which then causes structural alterations in the surrounding tissue. Cavitation leads to disordering of the lipid bilayers and formation of aqueous channels in the skin through which drugs can permeate and therefore increases the bioavailability of the drugs.
- (ii)
Thermal effects (increasing of temperature).
- (iii)
Induction of convective transport.
- (iv)
Mechanical effects (stress occurred because of pressure which is induced by ultrasound).
The experimental findings suggest that among all the ultrasound-related phenomena, evaluated cavitation has the dominant role in sonophoresis, which suggests that application of low-frequency ultrasound should enhance transdermal transport more effectively (Machet and Boucaud 2002).
Applications of ultrasound differ depending upon the frequency range of ultrasound used. For example, high-frequency (3–10 MHz) ultrasound is used for diagnostic conditions in clinical imaging, medium-frequency (0.7–3.0 MHz) ultrasound for therapeutic physical therapy and low-frequency (18–100 kHz) ultrasound for lithotripsy, cataract emulsification, liposuction, cancer therapy, dental descaling and ultrasonic scalpels (Gustavo et al. 2003).
There are innumerable applicators in the market that use sonophoresis technology. For instance, the ultrasonic teeth cleaning devices used by dentists have a frequency range of 25–40 KHz. Moreover, portable, pocket-size sonicators with rechargeable batteries for drug injection and analyte monitoring characterised with sensors are also available commercially (Mitragotri et al. 2000). In future applications, ultrasound technology seems to show promise for immunisation with vaccines and topical gene therapy. Some current applications consist of drug delivery, administration of targeted therapeutic and diagnostic agents, detection and determination of analyte, termination of cancer tissues, fatty tissues or kidney and gall bladder stones (Mitragotri et al. 1995).
It can be seen from various literature reports that ultrasound was used in an attempt to enhance the absorption of different molecules through the human skin. More recently, it was demonstrated that low-frequency ultrasound (<100 kHz), which causes cavitational disordering of SC, has been used to provide enhanced transdermal transport of low-molecular weight drugs, as well as high-molecular weight proteins (insulin, γ-interferon and erythropoietin) across the human skin (Mitragotri et al. 1995). There is good evidence for reversible effect on SC and potential usefulness of ultrasound within clinical settings (Mitragotri et al. 1996). But the commercial availability of ultrasound devices, especially for transdermal drug delivery, is very limited, even though many patents were filed. This represents that there is a need for more sophisticated devices, particularly addressing the advantages of low-frequency ultrasound for its non-thermal bioeffects, mostly by cavitations. Apart from the drug delivery applications, the novel noninvasive ultrasonic devices can conceivably be used in the extraction of clinically important analytes from the interstitial fluids of the skin. However, the successful application of these novel devices in drug delivery or monitoring needs to demonstrate successful preclinical or clinical studies before commercialisation.
17.2.1.6 Electroporation Microporation
Electroporation or electropermeabilisation is the temporary perturbation of structural lipid bilayer of biological membranes by the application of high-voltage pulses (≥50 V) and allows DNA or other macromolecules to enter the cells (Banga and Prausnitz 1998). When a short pulse is applied to generate a supra-breakdown potential across the membrane, the membrane will breakdown, leading to the creation of microchannels.
17.2.2 Physical Microporation Technique
17.2.2.1 MN Microporation
Apart from the various different techniques mentioned above, the microporation of the biological membrane, to desired depths, can also be achieved by the use of MNs (Fig. 17.1a). Even though, ALZA Corporation appears to be the first to use MN described in the late 1976 patent (Gerstel and Place 1976), the first paper to demonstrate MNs for transdermal delivery was not published until 1998 (Henry et al. 1998). MNs consist of plurality of microprojection arrays, generally ranging from 50 to 2000 μm in height, of different shapes and sizes. These MNs are attached to a base support, and simple physical application of such MN arrays to the skin surface can create transport pathways of micron dimensions in the biological membrane (Fig. 17.1b), unlike the above microporation devices that need external source of energy. MN can be solid or have a hollow bore and can be made from metal, polymers, elemental silicon or glass. For a detailed review on MN types, manufacturing technology and application in transdermal drug delivery in clinical studies, the reader is requested to refer relevant chapters in volume four of this book. Importantly, there has been a substantial increase in the attention that MN technology has received over the last 5 years, with a number of publications concerning MN evaluation more than tripling since 2005.
17.3 Safety Associated with the Use of Microporation Devices
Microporation of the skin results in breaching the skin’s SC barrier, thereby enhancing delivery of drugs of different physicochemical properties. However, application of microporation devices could also be associated with sensation of pain, erythema or both. Furthermore, by creating microchannels across the skin’s SC, its barrier property is compromised, whereby increasing the risk of invasion of exogenous materials (e.g. microorganisms), which depended upon the micropore dimensions. Additionally, multiple application of same microporation device can also pose the risk of contamination and could easily cause cross contamination between individuals. Furthermore, the nature of material used in fabrication of microporation device could also cause safety concerns, particularly if the material remains within the skin tissue due to improper use or accidental breakage during application. All the above concerns are integral component of these types of delivery systems and therefore need careful consideration. For example, in the past high-pressure jet devices have been employed for mass delivery of vaccination through the skin but fell from favour when a link to hepatitis B spread was established after vaccination with multi-dose injectors, which was caused by cross contamination between patients following jet application. This issue, in combination with the variability in patient response, such as occasional pain, discomfort and local reactions, inconvenience of use compared with injections and cost are potential barriers for the development and commercialisation of this drug delivery method (Hingson et al. 1963). Despite more than 50 years of clinical use and hundreds of patents, these jet injectors have not reached their full potential, in terms of replacing the routine needle-based delivery. However, recent demands of effective delivery of macromolecules including DNA (deoxyribonucleic acid), insulin, growth hormones, vaccines and other biotechnological products (Mitragotri 2006), in addition to other therapeutic drug molecules, have resulted in the improvement on the existing technologies of jet injectors. Especially, microjet injectors with low volumes, smaller nozzle diameters, and pulsed small injection volumes are considerably beneficial in reduction of occasional pain and consistence delivery. In addition, a better understanding of the jet injections on the skin at a cellular level, variability in jet penetration depending on skin properties, mechanisms of jet injections and mechanical properties of the skin need a special attention for effective performance. Likewise, RF-microchannelling devices and electroporation, which have shown, enhanced the delivery of both low- and high-molecular weight therapeutic molecules which are another promising technology. However, wider usage of such devices can only demonstrate the effectiveness of the technology and the suitability of devices.
On the other hand, the limited number of studies has demonstrated the use of laser-based microporation devices for the microporation of biological membrane; the possibility of portable laser devices will definitely improve the market potential transdermal drug delivery. However, more studies are required to demonstrate the clinical safety of using high-powered laser, the cost and its application for both drug delivery and minimally invasive monitoring.
The practicality of ultrasound microporation in health sciences as a biomedical applicator, as well as a therapeutic agent, is increasing. The use of ultrasound as an aid for increasing skin permeability depends upon the non-thermal bioeffects of its cavitation. In essence, attention should be paid to the issue of ultrasound technology’s effects on the structure of the skin to develop a useful tool that takes accounts for safety issues.
For all the microporation devices, a database of information on the microchannel closure rates should be provided, and factors affecting this should be thoroughly investigated, since the open micropore could jeopardise the skin’s barrier property.
17.3.1 Micropore Closure and Recovery Following Microporation Devices
Microporation devices can create transient aqueous microchannels, which enable transmembrane delivery of a wide range of molecules. But, creation of micron-sized pores or microchannels will also disrupt the SC barrier function of the skin. The degree of disruption is dependent upon the micropore dimensions, for example, in MN-based microporation technique, the needle of longer length causes greater barrier disruption than a needle of shorter length. However, irrespective of type of microporation method, the microchannels reseal over defined duration of time due to the skin’s natural repair mechanisms (Menon et al. 1992). Though significant the number of studies has been shown to improve drug delivery across microporated skin, very few studies have shown the characterisation of microchannels dimensions and its closure rates. In doing so, numerous instrumental methods have been reported to measure the rate of the skin’s resealing behaviour following application of microporation devices, such as dye staining, transepidermal water loss (TEWL) measurements, confocal microscopy, electrical impedance spectroscopy, histological staining and OCT. Noninvasive techniques such as TEWL measurements and digital imaging, following methylene blue staining (Fig. 17.3) of the microporated skin, are used as traditional techniques to determine the pore recovery or pore closure. However, more reliable and real-time in situ imaging techniques, such as OCT, have been demonstrated to give detailed information about the depth of penetration, dissolution (in the case of soluble MNs) and also skin recovery on a patient-to-patient basis (Fig. 17.4).
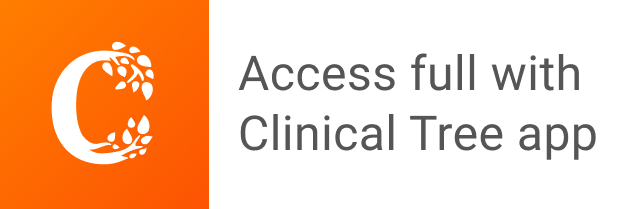