Fig. 4.1
Schematic illustration of skin (showing epidermis, dermis, hypodermis and skin appendages) and underlying muscles and joints, showing targets of drugs and cosmetics applied to skin for local, systemic or regional action
Chemicals are targeted to the aforementioned regions for therapeutic, cosmetic or diagnostic purposes, but unwanted contact of the skin with toxic and hazardous material can also occur. A good understanding of the skin barrier and the conditions that affect its barrier performance is essential for the development of therapeutic, cosmetic and diagnostic delivery systems and for minimising any unwanted effects.
Sources for skin responsiveness in addition to variations in skin permeability include the actions of metabolising enzymes and transporters in the various skin regions and the variation in target receptor populations in the various skin types. As shown in our recent review of the range and location of various metabolising enzymes and transporters in the skin (Dancik et al. 2010), many of the enzymes have either hydrolysis or oxidative type activities and include aryl hydrocarbon hydroxylase; 7-ethoxycoumarin O-deethylase; epoxide hydrolase; Cytochrome P450; esterases; aminopeptidases and peptidases. Hotchkiss (Hotchkiss 1998) suggests that the activities of cutaneous metabolising enzymes for Phase 1 metabolism (oxidation, reduction and hydrolysis) and for Phase 2 (conjugation) are 0.1–28 % and 0.6–50 % of those for the same enzymes in the liver. She also summarises the species and location variations for the various metabolising enzymes. As one illustration, the activity of cutaneous CYP1A1, a member of the Cytochrome P450 superfamily of enzymes, associated with the metabolism of benzo(a)pyrene and phenanthrene, is in the order SENCAR mouse > hairless guinea pig > human. The importance of location is illustrated in the work of Coomes et al. (1983), in which it is shown that for 7-ethoxycoumarin O-deethylase, benzo(a)pyrene hydroxylase (BPH), uridine 5′-diphospho- glucuronosyltransferase (UGT) and glutathione S-transferase (GST), the relative sebaceous cell to epidermal basal activities were: 6.0, 3,2, 2.9 and 3.5, respectively.
Importantly, as reviewed by Hotchkiss, the activity of all enzymes may be induced, inhibited or destroyed by various agents and processes (Hotchkiss 1998). For instance, Coomes et al. also showed that topical treatment of the skin with beta-naphthoflavone induced 7-ethoxycoumarin O-deethylase by more than tenfold for both sebaceous and basal cells, with much lesser effects on the other enzymes studied (Coomes et al. 1983). Finnen et al. reported variable induction of cutaneous 7-ethoxycoumarin O-deethylase by various corticosteroids (Finnen et al. 1984), while Lockley et al. have pointed out that the dermal exposure to glycol ethers can lead to skin sensitisation and irritancy as well as systemic toxicity as a result of their oxidation by cytosolic alcohol and aldehyde dehydrogenase (Lockley et al. 2005). Low concentrations (5 μM) of disulfiram inhibited skin cytosolic aldehyde dehydrogenase 1 (ALDH1)-oxidation of aldehydes from ethanol and glycol ether, whereas high concentrations (500 μM) led to complete inhibition. Ethanol, a penetration enhancer for the transport of beta-estradiol across the epidermis, is also an inhibitor of its cutaneous metabolism to estrone in the viable epidermis (Liu et al. 1991). In addition, the treatment of psoriasis and ectopic dermatitis patients’ skin with coal tar, a long-standing dermatologic treatment, for 24 h led to a two- to fivefold induction of aryl hydrocarbon hydroxylase (AHH) activity. Similar AHH induction was seen in excised normal human breast skin incubated with coal tar for 24 h (Bickers and Kappas 1978). Heating of full-thickness human skin at 60 °C for 2 min to separate the epidermis resulted in a 35 % reduction in phosphatase activity responsible for the hydrolysis of diisopropyl fluorophosphate (Loden 1985), while both freezing and heat separation led to a 40–50 % reduction in esterase hydrolysis of acetylsalicylic acid (Aspirin®, Bayer) in porcine skin (Lau et al. 2012). Cross et al. (1998) found that both full-thickness and separated epidermal membranes had methyl salicylate esterase activity that was about 25 % of the in vivo activity.
The effect of the metabolic activity on epidermal flux can be expressed as a first pass effect, whereby the flux of a solute entering the body after topical application is modified by the skin biovailability (F), i.e. the fraction of solute entering the body intact after topical application, calculated as the ratio of area under the plasma intact solute concentration-time profiles (AUC) after topical and intravenous dosing: F = AUC (topical) / AUC (iv). Wester et al. found the % bioavailability for nitroglycerin in rhesus monkeys to be 56.6 ± 5.8 % (Wester et al. 1983), while in healthy humans, it was estimated to be 75 % from Nitroderm® TTS patches manufactured by Novartis (Imhof et al. 1984) and 68–76 % from a transdermal ointment (Nakashima et al. 1987). As well as skin metabolism, the loss of nitroglycerin is due to retention in the skin and tissue binding (Imhof et al. 1984).
In the event of the complete loss of metabolic activity in in vitro skin, the steady state skin flux J s (= amount penetrated/time period) in vivo is related to that in vitro by Eq. 4.1 (Dancik et al. 2008):


(4.1)
4.3 Structure-Permeation Relationship in Percutaneous Absorption
Even though the advantages of transdermal delivery have been recognised for a long time, the drug candidates for application via this route are very few. The nature of the penetrant, in particular its physicochemical properties, always plays an important role in its passive delivery through the skin. Based on our knowledge of transdermal penetration and skin structure, it is generally accepted that the optimal molecular properties a drug candidate should possess for transdermal delivery are a low molecular size, a moderate lipophilicity, a low melting point and relatively few hydrogen bonding groups. Such requirements are discussed in more detail below. However, as we know, skin is not a homogenous barrier and contains a complex epidermal pathway, residing mainly in the stratum corneum, and an appendageal pathway, through hair follicles and other skin shunts, as illustrated in Fig. 4.1. In addition, structure-permeation relationship dependency relates to the nature of the barrier and it is difficult to find a general relationship to describe skin permeation overall. Besides considering a structure permeability relationship for permeation through the intact epidermis, physicochemical properties that favour a follicular pathway are also considered in this section.
Skin is a complex barrier, through which there are different parallel penetration routes via the intact epidermis and the appendages (hair follicles and sweat ducts) as well as serial barriers, including the stratum corneum, the viable epidermis, the dermis, etc. (Fig. 4.1). Even the stratum corneum (SC) contains parallel and serial pathways; the intercellular and transcellular routes. The SC is considered to be the main barrier to transdermal delivery in most cases. The transdermal permeation process, therefore, involves solute partitioning into and permeation through the appendageal pathway (App), partitioning into and permeation through the SC (transcellular or intercellular routes), partitioning between the SC and viable epidermis (VE), permeation through the VE, dermis and aqueous diffusion layers, and finally uptake by blood vessels or permeation through the hypodermis to underlying tissues [modified from (Zhang et al. 2009). This transport is expressed quantitatively, in its simplest form, as the steady state rate of permeation or flux (J s ) through the skin and is defined by the amount of solute (Q) passing through an area of skin (A) over a period of time (T) at steady state (i.e. T > an apparent lag time for permeation to occur and can be related to the maximum flux (J max) (that will be similar for all vehicles not affecting the skin) and fractional solubility in a given vehicle (f v ) (Roberts 2013) (Eq. 4.2), which, in principle, is related to the individual fluxes for solutes through the individual skin layers (stratum corneum, sc; viable epidermis, ve; dermis, de) and appendageal pathways (app) (Eq. 4.3):


As we will see later, dermal perfusion also affects the clearance of solutes from the dermis and the clearance of solutes across the dermis into deeper tissues, which, in turn, may affect viable epidermal concentrations. In this section, permeation of drugs through intact epidermis and the appendageal pathway will be discussed in relation to physicochemical properties of molecules.

(4.2)

(4.3)
4.4 Permeation Through Intact Epidermis
Considerable efforts have been made to establish a relationship between skin permeation and the molecular structure descriptors of solutes. The dependency of skin penetration on lipid-water partition coefficients, solubility, melting point and molecular size (molecular weight or molar volume) has been widely discussed in early studies published in this area (Scheuplein and Blank 1971; Lien and Tong 1973; Michaels et al. 1975; Roberts et al. 1977; Potts and Guy 1992; Du Plessis et al. 2002). In these studies, the SC was considered as the main barrier to permeation of drugs through the skin, but they recognised that the underlying viable epidermis, aqueous diffusion layer and dermis may also contribute to the skin resistance. Two main approaches have been used in defining these relationships: one is to express relationships in terms of observed fluxes through the skin and the other in terms of the physicochemical determinants often used to understand the variability in fluxes between different solutes and different formulations. The two approaches differ in that the first has its origins in the work of Higuchi (Higuchi 1960) and often relates permeation to the maximal permeation from a given vehicle, recognising that the flux of solutes from saturated solutions vehicles that have not affected the skin permeation should all be the same (Roberts 2013). In contrast, the second appears to arise from the conventions associated with epithelial permeability in biological systems where low solute concentrations are used. This approach defines transport in terms of a permeability coefficient; flux÷vehicle concentration (Kuswahyuning and Roberts 2014) and is limited by the variability in permeability coefficient between vehicles, in accordance with the variation in solute solubility in the different vehicles (Roberts 2013). Care should be applied when using such relationships, as most vehicles do in fact affect skin permeability, they may affect dermal blood flow, and solutes could be in solution in a supersaturated form, i.e. above the usual solubility of the solute in the vehicle and therefore be associated with higher fluxes. The use of saturated or maximum flux has the advantage of directly showing the maximum dose deliverable over a period of time (Magnusson et al. 2004; Sloan et al. 2006; Milewski and Stinchcomb 2012). Further, if neither the formulation nor the solute affects the membrane, it should be an invariant in the thermodynamic description of the penetration process, whereas the permeability coefficient depends on the formulation used (Zhang et al. 2009; Scheuplein 2013). However, a changed maximum flux after formulation application may suggest an alteration of the skin bioproperties by the components of the vehicle, either during solute partitioning or the diffusion process.
Relevant to the first approach, we showed that the main determinant for maximal (or more accurately, saturated) solute flux from aqueous vehicles is the solute size (molecular weight or molecular volume) with an r 2 of 0.688 (n = 278, p < 0.001) (Magnusson et al. 2004):

A limitation of Eq. 4.3 is that it really only reflects the effect of diffusivity on skin transport, noting the diffusivity is a function of solute size, as defined by free volume and other theories (Kasting et al. 1987). It does not reflect the contribution of solubility of solutes to the flux. The theoretical importance of solubility is evident when it is assumed that the SC is the main barrier for solute penetration and when the saturated solute flux in expressed terms of its underlying determinants for skin penetration: diffusivity in the SC (D sc), the path length for transport across the SC (h sc) and the solubility in the SC (S sc). Hence, incorporating these into Eq. 4.1 and expanding by recognising that the SC-water partition coefficient (K sc,v ) is given by (S sc/S v ) yields:

Thus, it is evident from Eq. 4.5 that the solubility of the solute in the SC, S sc, can be expressed in terms of a solubility of the solute in the vehicle and a partition coefficient K sc,v between the SC and vehicle (i.e.
) and, in turn, the permeability coefficient is the product of this partition coefficient multiplied by D sc/h sc (i.e.
). We have previously applied
and the relationships between log K sc and log P (Roberts et al. 1996), the dependence of solubility of the solutes in water on the octanol-water partition coefficient and the solute melting point above 25 °C (Yalkowsky and Valvani 1980) to yield (Roberts et al. 2002):

Thus, the overall saturated flux is given by a combination of Eqs. 4.3 and 4.4. A difficulty in this area is the accuracy of the predictions for both SC-water partition coefficients and in water solubility of different solutes. The most recent relationship, recognising most of the previous studies, suggests the slope for the linear relationship for a large data set of lipophilic solutes is 0.69 (Wang et al. 2010), slightly higher than the slope of 0.57 we found for our data set. Hewitt et al. (2009) recently evaluated the generalised solubility model used by Yalkowsky and colleagues (Yalkowsky and Valvani 1980) and commented: “The final conclusion of this study must be that predicting aqueous solubility is indeed still a formidable challenge! The results obtained in this study clearly indicate that no one model was able to predict solubility accurately.” Indeed, Ali et al. (2012) are also advocating the inclusion of the total polar surface area in estimation of aqueous solubility for some solutes. Magnusson et al., in recognising SC solubility as a determinant of flux, showed that inclusion of melting point (MP) and hydrogen bond acceptor ability increases the r 2 for the prediction of saturated fluxes (Eq. 4.3) to 0.760.

(4.4)

(4.5)




(4.6)
The effect of solubility will be most evident for a group of solutes that are approximately similar in size (i.e. similar diffusivity). We and others have shown that there is a parabolic relationship between the saturated solute flux for such solutes and log P (a measure of solute polarity) (Yano et al. 1986; Hinz et al. 1991; Magnusson et al. 2006; Zhang et al. 2009). Several other studies have shown a parabolic relationship between maximum flux and solute lipophilicity in vitro in humans (Cross et al. 2001), hairless rat (Diez et al. 1991) and hairless mouse skin (Hinz et al. 1991) and in vivo (Yano et al. 1986; Bucks and Maibach 2005). Parabolic relationships seen in our data (Zhang et al. 2009) with epidermal membranes and that of Yano (humans in vivo) and Hinz (hairless mouse skin) are illustrated in Fig. 4.2.


Fig. 4.2
Convex dependence on log P seen in (a) log Jmax a series of similar-sized phenols (MW approx. 150) applied in aqueous solutions to excised human epidermal membranes. (Original data from Zhang et al. 2009). (b) log (% absorption over 4 h) for salicylates (closed symbols) and non-steroidal anti-inflammatory drugs (open symbols) applied to human skin in vivo. Original data from (Yano et al. 1986) and (c) log J max for a series of phenols across hairless mouse skin in vitro (Original data from (Hinz et al. 1991). The curves are for illustrative purposes only
We also showed a parabolic relationship between the SC solubility and log P for phenols and that the saturated flux versus SC solubility was linear (Fig. 4.3), with a log P independent diffusivity for these similar size solutes, showing that this saturated flux – log P relationship was defined by a variation in SC solubility with log P.


Fig. 4.3
Relationships between maximum flux (Jmax, estimated from a 10 % solution), stratum corneum solubility (S sc) and log P (experimentally determined) for a series of similar-sized phenols applied in aqueous solutions to excised human epidermal membranes. (a) log J max vs log P; (b) log S sc vs log P; (c) linear relationship between log J max and log S sc (Data adapted from (Zhang et al. 2009). The curves are for illustrative purposes only
We then extended this study by using a propylene glycol-water co-solvent vehicle (Zhang et al. 2011), concluding that propylene glycol containing vehicles enhanced the maximum flux of these solutes by increasing their solubility in the intercellular lipids of the SC, but again showing that diffusivity was not dependent on the lipophilicity of the solute.
The second approach is to examine skin permeation in terms of the skin permeability coefficient (k p ), which is defined by two main parameters: diffusion and partition coefficients (Eq. 4.5). Traditionally, these have been represented by a combination of organic – water partition coefficients (presented by log P) and size (represented by molecular weight MW or molecular volume MV) (Lien et al. 1971). The most comprehensive analysis of k p in terms of solute size and lipophilicity was presented by Potts and Guy (Potts and Guy 1992), as shown in Eq 4.7:

Many others have since attempted to improve estimation of k p (Lian et al. 2008; Chen et al. 2010; Moss et al. 2012). In a recent work, Milewski and Stinchcomb (Milewski and Stinchcomb 2012) have arrived at a predictive model for saturated skin fluxes by combining the Potts Guy (Eq. 4.7) with that from the Yalkowsky group (Yalkowsky and Valvani 1980) for water solubility to yield Eq. 4.8 for estimation of J s,sat from log P, MW and MP (n = 208, r 2 = 0.81).

Whilst this model probably is currently limited to aqueous solutions, it may be applied to other vehicles if the concentration of the solute in the vehicle can be expressed as a fractional solubility in a simple or complex vehicle, Raoult’s Law is approximately obeyed and the vehicle has either not affected the skin or its extent of skin permeation enhancement is known. We have pointed out that when the fractional solubilities for the vehicle
and skin
can be assumed to be equal, the skin flux is given by Eq. 4.1, i.e.
(Roberts 2013). Despite much effort in developing quantitative structure–activity relationship (QSAR) models, the predictability and quality of these models for risk assessment purposes can be further improved. A key issue is the large set of historical experimental data, in which variability is likely to have arisen from the use of different experimental approaches and human skin donors. In an attempt to cover as many formulations and conditions as possible, Samaras et al. (Samaras et al. 2012) examined the in vitro skin fluxes from the EDETOX database in their regression analyses in terms of not only the molecular descriptors of the penetrants and the vehicle, but also the range of various experimental and vehicle conditions used – including finite/infinite dosing, skin pre-hydration, occlusion and the skin thickness. They concluded that the donor concentration, solute lipophilicity, solute size and solute polarity, as well as the vehicle melting and boiling points were prominent factors influencing skin flux.

(4.7)

(4.8)



In reality, the SC is a heterogeneous membrane consisting of several layers of corneocytes with the space filled with lipids. Some hydrophilic and polar solutes penetrate through the SC faster than would be anticipated based on lipophilicity alone (Nitsche et al. 2006; Wang et al. 2007; Mitragotri et al. 2011). Consequently, the “porous pathway” model was introduced to address this challenge by describing skin permeability to hydrophilic solutes (Peck et al. 1994; Lai and Roberts 1999; Tang et al. 2001; Tezel et al. 2002; Mitragotri 2003). In this model, hydrophilic solutes are assumed to diffuse through pores in the skin, where pore size distribution, skin porosity and tortuosity are considered. The “brick-and-mortar” model has also been adapted and expressed in different forms (Wang et al. 2006, 2007; Chen et al. 2010, 2013). This mathematic model aims to include permeation through the corneocytes (i.e. transcellular pathway) and intercellular lipids (intercellular pathway), where the geometrical and compositional parameters of the SC have been included.
4.5 Physicochemical Properties That Favour Appendageal Permeation
As an alternative to the stratum corneum inter- and intracellular permeation routes, the “bulk pathway” or “shunt pathway” by appendages such as hair follicles also acts as a permeation route through the skin (Fig. 4.1). This pathway has previously been considered as insignificant as appendageal structures only make up 0.1–1 % (forearm to forehead) of the total skin surface area (Otberg et al. 2004). However, in recent years, hair follicles and their associated pilosebaceous structures acting as a permeation pathway and/or reservoirs have been increasingly explored and received more attention, especially for nanoparticle delivery (Lademann et al. 2008; Patzelt and Lademann 2013).
The pilosebaceous unit comprises four different areas for topical application: the sebaceous gland, the bulb region, the hair matrix cells and the hair follicle infundibulum. Sebum excreted by the sebaceous gland is composed of short-chain fatty acids, resulting in a microenvironment that is rich is neutral, non-polar lipids (Meidan et al. 2005). The lower infundibulum provides decreased barrier properties and is surrounded by a dense network of blood capillaries. The infundibulum increases the possible surface area for targeted delivery and is an area of additional absorption. The immediate region surrounding the infundibulum is rich in antigen-presenting cells, whereas the lower bulge region is surrounded by stem cells (Patzelt and Lademann 2013), providing target sites for penetrated molecules (Knorr et al. 2009).
Work by a number of groups has found that the follicular route predominates for hydrophilic molecules (Essa et al. 2002; Ogiso et al. 2002; Mitragotri 2003) that cannot permeate the epidermal pathway easily. A study by Frum et al. (2007) quantified the influence of the drug lipophilicity on follicular penetration by using a range of drugs with similar molecular weights. A parabolic profile shows a maximal follicular contribution occurs at log P around 1. The two most lipophilic drugs, estradiol and corticosterone, had minimal entry into the follicular pores, whereas a 34 % contribution to total skin flux was found for hydrophilic adenosine (log P = −1.05). For mannitol, which is also hydrophilic (log P = −2.47) there was a 54 % follicular contribution associated with its flux through human skin, though its molecular size is slightly smaller than the above penetrants (Essa et al. 2002).
The in vivo follicular penetration of caffeine was also systemically examined using pharmacokinetic modelling (Liu et al. 2011). There was a 10 times faster absorption from hair follicles, and a 10-min delay for transport through the stratum corneum, with the follicular route contributing 25–35 % to the overall skin absorption. The in vivo absorption of caffeine was fast and high compared to the in vitro absorption, and this was considered to be mainly due to a fast resorption by in vivo blood flow, the physiological difference in hair follicles of the investigated skin and a contraction of hair follicles of the excised skin.
Besides polar molecules, the transfollicular pathway is also an important route for nanoparticles, which may be targeted to deep follicular regions and function as drug delivery vehicles (Lademann et al. 2007). Topically applied polymeric particles were shown to penetrate porcine skin with massage via the follicular pathway in a size-dependent manner (Patzelt et al. 2011), with 643-nm particles penetrating deepest into the follicle, to a maximum depth of approximately 1100 μm (see Fig. 4.4).


Fig. 4.4
Above: Penetration depths in μm of different sizes of PLGA particles in relation to the target sites within terminal hair follicles (THF) and vellus hair follicles (VHF). Below: The hair surface structure, showing the layers of overlapping cells (cuticula pili) (Reproduced with permission from Patzelt et al. 2011 and Lademann et al. 2011)
Lademann has suggested that the optimal size of particles for deep penetration is governed by the size of the cuticula on the hair shafts (Lademann et al. 2011) (see Fig. 4.4), which may act as “geared pumps” to drive the particles into the follicles during massage (Lademann et al. 2009). The effect of massage is demonstrated in Fig. 4.5, where both particles and dye molecules remain in the superficial regions of the follicle without massage, whereas, massage drives the particles – but not the dye – deep into the follicle (Lademann et al. 2007).


Fig. 4.5
Superposition of transmission and fluorescent images, demonstrating the in vitro penetration of the dye-containing formulation into the hair follicles of porcine skin after application of massage. (a) Dye in particle form and (b) dye in non-particle form – with massage. (c) Particle form and (d) non-particle form – without massage (Reproduced with permission from Lademann et al. 2007)
Particles, which may be solid or vesicular, with therapeutic substances bound to the surface or encapsulated, may be ideal follicular delivery agents, because of their long residence time within the follicle and their targetable penetration depth (Lademann et al. 2008). In addition, Vogt et al. found that antigen-presenting cells surrounding the infundibulum are able to internalise 40-nm particles that have permeated through the follicular epithelium (Vogt et al. 2006), thus providing a promising mechanism for vaccination via the follicular route.
Targeted follicular delivery can be achieved by manipulating the formulation (i.e. particle-/vesicle-based dosage forms and using sebum miscible ingredients) or modifying the target molecule (optimising physicochemical properties such as size, lipophilicity, solubility parameters and charge) (Trommer and Neubert 2006). An example reported by Grice et al. (2010) shows that formulations that contained ethanol could enhance the early minoxidil uptake into the hair follicles. As minoxidil is six times more soluble in ethanol as it is in water, this was likely due to the partitioning of ethanol (plus minoxidil) through the lipid rich compartments. Some other methods like fractional laser and massage could also selectively enhance permeant targeting to follicles (Lademann et al. 2007; Lee et al. 2013).
The importance and required molecular and particular properties for an optimum follicular delivery are yet to be fully investigated.
4.6 Physicochemical Properties That Favour Direct Subcutaneous and Deeper Tissue Permeation
There are now a large number of studies showing that deep tissue penetration can occur after topical application to specific areas of the body. Various human biopsy (Rabinowitz et al. 1982; Grundmann-Kollmann et al. 2002; Anissimov and Roberts 2011) or cutaneous microdialysis (Cross et al. 1998; Benfeldt et al. 1999; Brunner et al. 2005) studies have been used to show direct and deep tissue penetration of solutes after topical application.
Rat wound deposition studies have been used to show that the main determinant for the penetration of solutes into deeper tissues after topical application is the size of the solute (Singh and Roberts 1996; Cross and Roberts 1999; Kretsos et al. 2008). The cutaneous vascular appears to be implicated in the penetration of solutes in studies conducted in rats, pigs and man (McNeill et al. 1992; Monteiro-Riviere et al. 1993; Cross et al. 1999).
Dancik et al. (2012) combined experimental percutaneous permeation studies with the available microdialysis literature on the penetration of topically compounds into subcutaneous tissue and muscle. The solutes studied were of similar size and included diclofenac, ibuprofen, fluconazole, lidocaine, nicotine, propranolol, ethanol, 5-fluorouracil, methyl salicylate, salicylic acid, salicylic compounds and trolamine salicylate. They observed that, in general, the concentration gradients for highly protein bound drugs for deeper tissue penetration tended to be shallow, whereas the poorly bound drugs showed an exponential concentration gradient. They applied a physiological pharmacokinetic analysis to this data and concluded that highly plasma protein bound drugs, in addition to normal diffusive transport, may be transported in deeper tissues via the blood and lymphatic circulation as well as via interstitial convection (Fig. 4.6) and that these transport routes may dominate in some cases. Such vascular transport can increase deep tissue concentrations beyond those resulting from extravascular diffusion alone, and decrease the drug’s lag time into deep dermis, subcutaneous and muscle tissue.


Fig. 4.6
Physiological pharmacokinetic model for topical drug transport processes in deeper skin. (a) Blood vessels, lymphatic vessels and interstitial convection in dermis. (b) Schematic of physiological pharmacokinetic model used to analyse human cutaneous microdialysis data at two depths, the concentration–time profile in the superficial microdialysis probe being used as an input to predict the deeper microdialysis probe concentration–time profile (Reproduced with permission from (Dancik et al. 2012))
4.7 Skin Hydration and Percutaneous Absorption
Transdermal delivery can also be controlled by the extent of skin hydration, which mainly refers to the extent of hydration in the SC. A major controller of hydration is natural moisturising factor (NMF). The original bricks and mortar description of the SC has been refined substantially and it is now described as being a continuous poly-proteinaceous structure, with the bricks being likened to NMF-containing keratin sponges. NMF functions as a plasticiser of the stratum corneum under basal conditions (Laden 1967; Jokura et al. 1995). Following the addition of water or under conditions of high humidity, the NMF-bound water assists in the swelling of the corneocytes. Swelling is at its greatest in regions where the NMF concentration is at its highest (Bouwstra et al. 2003). It has been shown that when the skin is highly hydrated, water is predominantly located either in the intercellular regions in separate domains or trapped in corneocytes (Roberts et al. 2008). In addition, swollen corneocytes and separation of lipid bilayers in the intercellular space to form cisternae and networks of spherical particulates are found in porcine skin after a 4–10-h hydration period (Tan et al. 2010). Figure 4.7 shows high magnification cryo-scanning electron microscopy images of human SC in various hydration states, showing clearly the swollen corneocytes and water pools present at high levels of hydration (Bouwstra et al. 2003).


Fig. 4.7
High magnification cryo-scanning electron microscopy images of SC hydrated to various levels. Arrows indicate undulations or interdigitations of cells. Arrowheads indicate the cell boundaries. (a) Dry SC characterised by low contrast images. The cells are approximately 360 nm in thickness. The spaces between the corneocytes are mostly air-filled, possibly caused by drying of the SC required for low hydration levels. Arrows indicate undulations or interdigitations of cells. Arrowheads indicate the cell boundaries. T, tissue-freezing medium. (b) SC hydrated to 17 % wt/wt reveals a low contrast image, similar to that observed for dry skin. This indicates the absence of water pools. (c) SC hydrated to 70 % wt/wt reveals in the central part slightly swollen cells with a higher contrast (see black asterisks) indicating the presence of water. In the upper and lower part, the appearance of the SC is similar to that of dry skin (white asterisks). (d) A high magnification of a fully hydrated SC sheet. The keratin network is clearly depicted. This network is surrounded by the cornified envelope. Between the cells frequently large and very small water pools are observed (see white arrows). If no water pools are present a close cell to cell contact is observed. The cell ends are round and fewer undulations are observed compared to dry SC or SC hydrated to approximately 20 % wt/wt. A difference in the degree of swelling between the various cells is noticed. The cells that are less swollen are indicated by short arrows (Reproduced with permission from Bouwstra et al. 2003)
The hydration state of the stratum corneum is mainly influenced by environmental humidity, temperature, transepidermal water loss (TEWL) rate, keratinisation rate and the type of moisturisers (Vergnanini et al. 2010). The inverse relationship between TEWL and SC hydration is well known, just as disturbed skin barrier function has been correlated with low SC hydration (Proksch et al. 2008).
In the presence of an intact barrier, increasing the water content of the skin can have substantial effects on the transdermal delivery of penetrants by altering skin physiology. In clinical practice, occlusion of the skin, by which the hydration of the SC can be greatly increased, is widely used to enhance the penetration of topically applied drugs (Hafeez and Maibach 2013). A number of factors could contribute to the increased permeability. Firstly, it is likely to be due to an increase in the solubility of the permeant in the SC. This increases the partitioning of the solute from the vehicle into the membrane. Another possibility is that the increased hydration could lead to swelling of the structure and rearrangement of the lipids. Recent work using Raman spectroscopy demonstrated that increased amounts of unbound water in the stratum corneum, also illustrated by cryo- electron microscopy (Fig. 4.7) could disorder the structures of lipids and proteins (Vyumvuhore et al. 2013), thereby altering the permeation rate of topically applied solutes. Changes in skin hydration may affect skin permeation by several orders of magnitude as previously described (Roberts 1991; Roberts and Walker 1993). Evidence shows that increasing room humidity can lead to a marked increase in absorption for a range of solutes including aspirin, fusidic acid, methylethylketone, various corticosteroids, etc. (Roberts and Walker 1993).
Interestingly, current findings suggest that there are no quantitatively defined relationships between hydration-enhanced skin permeability and the lipophilicity and molecular structure of the solutes, although the early study by Wurster and Kramer (1961) suggested that hydration preferentially enhanced the skin penetration of the more polar salicylate esters.
Taylor et al. (2002) found that occlusion of the skin affected the skin penetration of linoleic acid and glycerol differently, with occlusion suppressing the delivery of linoleic acid from an ethanolic vehicle compared to the unoccluded condition, but with little influence on the percutaneous absorption of glycerol. Work by Hikima and Maibach (2006) showed a random distribution in human skin fluxes of steroids in relation to their lipophilicity or molecular weight when the skin was either hydrated or dehydrated. A similar result was found by Bucks and Maibach (2005), where a series of phenolic compounds with varying lipophilicity was examined. In the same study, the more lipophilic steroids appear to have a greater enhancement by occlusion than the more polar ones (Fig. 4.8).


Fig. 4.8
Occlusivity does not uniformly enhance penetration of solutes with varying octanol water partition coefficient (log P) across human skin in vivo: (a) Phenols, (b) steroids; open symbols occluded, closed symbols unoccluded (Data modified from (Bucks and Maibach 2005))
It should be noted that the effects of occlusion on skin permeation are not clearly defined or uniform (Roberts et al. 2004; Pellanda et al. 2007). For example, Cevc et al. (2008) found that skin occlusion suppressed the permeation of ketoprofen delivered from ultra-deformable, hydrophilic transfersome carriers by apparently eliminating the transcutaneous water gradient that normally drives the transfersomes across the skin. In contrast, the permeability of topically applied free ketoprofen was promoted by occlusion. Samaras et al. (2012) further showed that skin occlusion had a significant non-linear effect in their statistically valid QSAR developed from an extended database. Hafeez and Maibach (2013) recently concluded that occlusion was more effective in enhancing permeation of very lipophilic compounds than in relatively hydrophilic compounds, but emphasised that no clear relationship between lipophilicity and occlusion-induced permeation enhancement could be discerned.
Occlusion is not the only means of increasing skin hydration. Chemical and physical enhancement methods can also affect skin hydration. For instance, iontophoresis may hydrate the stratum corneum due to the formation of water pools in the intercellular regions (Fatouros et al. 2006). Water-binding agents (humectants and or hydrating agents) are also classified as moisturisers that prevent water loss and have a softening and soothing effect on the skin. For example, urea, an ingredient in some hair conditioners, facial cleansers, bath oils, skin softeners and lotions, has good water-binding and exfoliating properties for skin when used in small amounts. Some studies have also shown that urea may act as a penetration enhancer (Wohlrab 1984, 1990; Beastall et al. 1986). Glycerin based creams, very familiar to most consumers, also have humectant properties. Humectants with a small molecular size (e.g. urea and glycerin) are assumed to penetrate into the stratum corneum, mimicking the role of natural moisturising factors to retain moisture (Caussin et al. 2007). However, the mode of action is still poorly understood (Loden 2008).
4.8 Age-Related Skin Barrier Performance
Epidermal development, including SC structure, is complete in utero by 34 weeks. Pre-term babies have elevated levels of both TEWL and transcutaneous heat transfer, which as expected, are largely dependent on how premature the baby is. Ultra-low birth weight infants from the ages of 23–25 weeks were shown to need at least 4 weeks to develop a fully functional SC, whereas babies of 30–32 weeks had barrier functions comparable to adults (Kalia et al. 1998; Fluhr et al. 2004).
It has been widely reported that premature infant skin is more susceptible to penetration than fully formed infant skin. A study by Anderson et al. showed that when hexachlorophene was used as a disinfectant on the skin of pre-term infants, dermal absorption occurred which resulted in myelinopathy in the premature infants (Anderson et al. 1975). Another study by Barker et al. examined the permeation of sodium salicylate through ex vivo newborn infant skin. It was found that absorption of the compound was 100–1000 times higher in infants who had a gestation period of 30 weeks or less, compared to full term infants (Barker et al. 1987).
Giusti et al. measured the TEWL, capacitance and pH of the volar forearm and buttocks of 70 infants aged 8–24 months and compared these to values in adult skin (Giusti et al. 2001). As expected, no differences in TEWL were found between the infants and adults at either site, confirming that the infant skin had a fully developed barrier function. Capacitance values, which quantify the amount of water in the SC, were found to be higher in infants, as were the pH readings. No TEWL, capacitance or pH variations were observed in infants according to sex or age.
As the skin ages, certain structural changes occur, so that the aged SC is considerably drier than that of a young adult, it has a reduced lipid content and frequently has a less extensive microcirculation. The epidermis becomes thinner and the keratinocytes become less adjacent to one another. The dermis also becomes relatively atrophic, acellular and avascular. Collagen, elastin and glycosaminoglycan are also altered. Exactly how the penetration barrier of the skin changes with age remains unclear; however, it should be assumed that aged skin provides a more variable environment for the diffusion of topically applied drugs, as discussed below (Byl 1995).
Waller and Maibach reviewed the literature on the effect of aging on blood flow, pH, thickness and ultrasound echogenicity (Waller and Maibach 2005). They concluded that the data which described age-related changes in skin structure and function were often conflicting and difficult to interpret. The cutaneous blood flow results suggested that there may be a trend towards decreased cutaneous perfusion in older individuals, especially in photo-aged areas. The skin pH data were more convincing, as it appears that skin pH is constant throughout adulthood to approximately 70 years of age. After 70, the pH increased, which was suggested to be due to stasis of blood, especially in the lower limbs. The change in thickness of the skin layers seemed to have been influenced more by site on the body than by age. The epidermis thickens with age on certain sites such as the volar and dorsal upper arm, but remains constant in areas such as the buttock, dorsal forearm and shoulder. Similar variations were noted for dermal thickness.
TEWL has been used as an indication of the effect of aging on barrier function. Hillebrand and Wickett noted considerable variability in TEWL with age in Chinese female women living in Beijing, with a quadratic regression showing little change between 10 and 70 years (Hillebrand and Wickett 2008). They point out that an alternative explanation for these findings is that the women in the different age groups experienced different stresses (sun, diet, exercise) depending on where they were born, which may dominate any relationship between TEWL and skin age. In another study of adults aged 19–85 years showing no correlation of ventral forearm TEWL with age, Roskos and Guy concluded that the ability of the SC to control evaporation from the skin is not age-dependent and its ability to prevent dehydration is also maintained as a subject ages (Roskos and Guy 1989). When the SC barrier was deliberately perturbed by occlusion, the water activity gradient was lost more rapidly in the younger group (age 19–42) than the older group (age 69–85). The time it took for water to diffuse through the SC was longer for older patients. It was explained that when the tissue was occluded, less water may have been required for the SC to achieve the fully hydrated equilibrium state. As older skin has been shown to have reduced water content, it requires more water to achieve a fully hydrated state. It is possible that occlusion in the older subjects produces a hydration-induced structural alteration of the intercellular lipid organisation. The subsequent dehydration (and reorganisation of this structural modification) could manifest slower release kinetics. Similar findings of a minimal effect of age on TEWL at six different body sites in women were reported by Luebberding et al. (2013). In the same study, significant decreases in sebum production were seen with increasing age, with the lowest skin surface lipid levels seen in subjects over 70 years old.
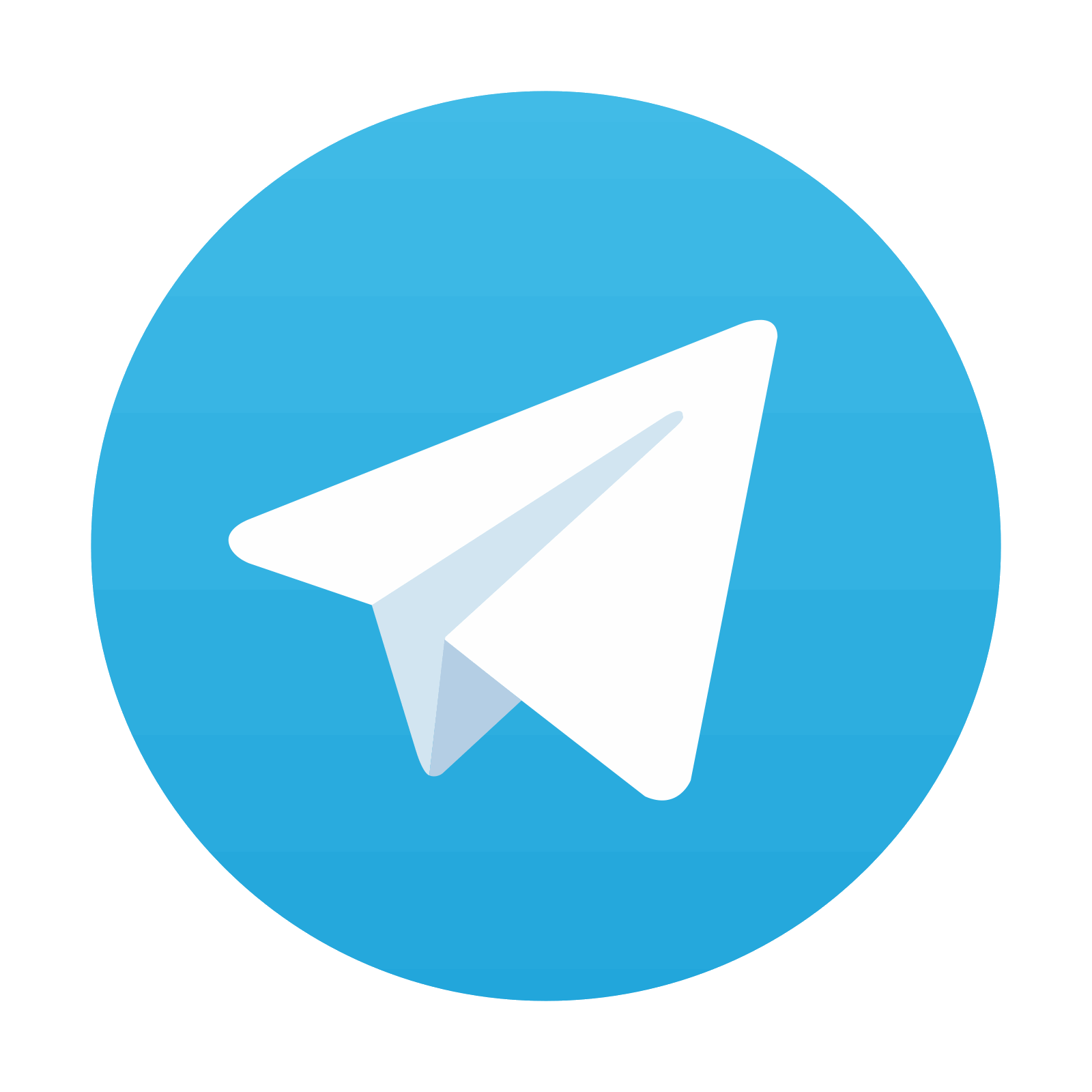
Stay updated, free articles. Join our Telegram channel
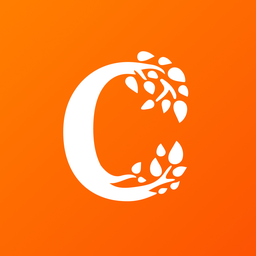
Full access? Get Clinical Tree
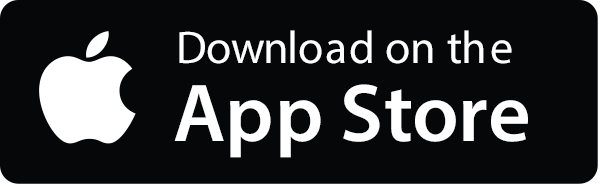
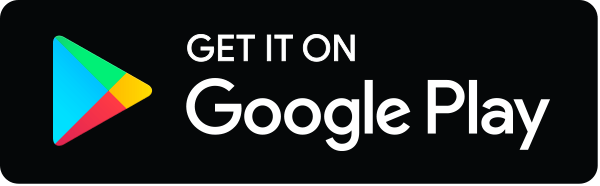