Introduction and Historical Notes
The peripheral nervous system (PNS) embodies unique anatomical structure and function that contributes immensely to human quality of life. Through the ages, the importance of the PNS has been recognized, but function and structure were often misinterpreted or considered mystical. The Alexandrians, specifically Herophilus of Chalcedon (335–280 bc ) and Erasistratus of Chios (310–250 bc ) are credited with differentiating nerves from tendons and even proposed the presence of different nerves for motor and sensory functions. Erasistratus distinguished nerves, in addition to arteries and veins, as one of the three main channels in the body, but proposed that “animal spirits” flowed through the nerves, subserving sensation and movement. The electrical basis of nerve and muscle potentials was better realized after Arrhenius (1859–1927) and other physical chemists demonstrated the properties of ions in electrolyte solutions, and nerves and muscles were found to conform to membrane potentials predicted by the Nernst equation. Technological advances during the First World War allowed revelation of the properties of nerve action potentials. Continued advancement of our knowledge of the structure and function of the PNS, as well as the instruments to study it have allowed us to not only better understand PNS pathology, but intricately modify and repair it to better improve the human experience.
Anatomical Considerations
The PNS is composed of neurons and supporting glial cells called Schwann cells . Neurons may be motor, sensory, or mixed. The neuron cell bodies are located in the spinal cord, and long cytoplasmic peripheral extensions called axons carry electrical impulses to or from their targets. Axons are separated from one another via endoneurium, and organized groups of axons are called fascicles ( Fig. 24.1 ). The perineurium surrounds fascicles, and the internal epineurium separates the perineurial-ensheathed fascicles. The external epineurium encompasses all fascicles and the internal epineurium and establishes the nerve silhouette. The mesoneurium is located external to the epineurial layers, is continuous with the perineurium, and is critical in longitudinal gliding of the nerve. Schwann cells (SCs) are subdivided into two main types: myelinating SCs and non-myelinating SCs. Myelinating SCs insulate and protect axons by iteratively wrapping their membranes to form the multilaminated myelin sheath, ensuring that nerve impulses travel quickly and efficiently. Independent of their functions in myelination, Schwann cells also provide vital trophic support to neurons. Loss of myelin can lead to permanent neuron loss, significant pain, and morbidity, and ultimately paralysis. Non-myelinating SCs are located along axons and at the neuromuscular junction and provide many functions including trophic support, phagocytosis, synaptic transmission, and support for reinnervation. More knowledge regarding the importance of non-myelinating SCs is evolving constantly.

Fascicular nerve patterns may be monofascicular with just one large fascicle; oligofascicular , consisting of a few fascicles; and polyfascicular, consisting of many fascicles of varying sizes. In the proximal extremities a monofascicular pattern predominates, consisting of both motor and sensory nerves with considerable plexus formation among fascicles. Moving more distally, the plexus formation simplifies and a more specific, polyfascicular pattern with differentiation into motor and sensory regions closer to the end-target, is found.
Knowledge of internal nerve topography allows specific fascicular alignment during repair to enhance efficiency and specificity of recovery. Topography for specific nerves has been described and results from intraoperative electrical stimulation to differentiate motor and sensory regions. Nerve transfers, such as the double fascicular transfer, are a direct application of the knowledge of internal topography.
The vascular supply for nerves is via two main systems, collectively referred to as the “vasa nervorum.” The mesoneurium provides the extrinsic vascularity, while the endoneurium houses the intrinsic nerve vascular supply. The extrinsic supply was described by Ranvier as early as 1878, upon which Ramage further elaborated. The intrinsic supply consists of fragile capillary networks that are easily disrupted. Vasa nervorum have been described in detail for specific nerves. The vasa nervorum are linked to injury and repair. Flow is increased in the extrinsic and intrinsic systems in the acute period following injury with angiogenesis occurring in the perineurium and epineurium approximately 2 weeks after injury in the rat model. After prolonged denervation (>6 months), vascular flow is diminished, and this phenomenon reverses after reinnervation.
Classification of Nerve Injury
Correct classification of nerve injury is paramount to planning appropriate management strategies. Establishing the degree of nerve injury predicts prognosis for recovery or lack thereof. In 1943, Seddon initially categorized nerve injury into neurapraxia, axonotmesis, and neurotmesis. This classification system was later elaborated by Sunderland in 1951, into five degrees of nerve injury, and he alluded to possible combinations of these degrees of injury. Mackinnon popularized the mixed pattern, or sixth degree, of injury, which describes multiple injury patterns at the same level ( Table 24.1 ).
Degree of injury | Recovery | Rate of recovery | Surgical management | Electrodiagnostic studies | |
---|---|---|---|---|---|
Favorable | |||||
I | Neurapraxia | Complete | Up to 12 weeks | − | No fibrillations |
II | Axonotmesis | Partial + | 1 mm daily | ± | Fibrillations and MUPs |
III | Axonotmesis | Partial | 1 mm daily | ± | Fibrillations and MUPs |
Unfavorable | |||||
IV | Neuroma-in-continuity | None | None | + | Fibrillations, no MUPs |
V | Neurotmesis | None | None | + | Fibrillations, no MUPs |
VI | Mixed injury (I–V) | Some fascicles (II, III) | Varies with injury (I–V) | + | Varies with injury |
Both first- and second-degree injuries can be grouped into favorable injuries, are expected to recover spontaneously, and therefore are typically managed conservatively. A first-degree injury, or “neurapraxia,” is an ischemic injury or conduction block which may have segmental demyelination, but no interruption of axons or surrounding connective tissue. As a result, no axonal degeneration occurs, and complete recovery is expected within 12 weeks after axonal remyelination is complete. In cases of chronic nerve compression, a permanent conduction block may ensue. Second-degree injuries, or “axonometric injuries,” involve axonal disruption but persistent continuity of surrounding connective tissue sheaths, including the basal lamina. The axons distal to the injury site undergo Wallerian degeneration, and recovery is expected in alignment with nerve regeneration at a rate of 1 mm daily. Motor nerve injuries distant to the target muscle may be at risk for incomplete recovery due to the deleterious microenvironment occurring with prolonged denervation in both the nerve and target muscle.
More severe peripheral nerve injuries require surgical intervention. Third-degree injuries are also axonometric injuries, with injury to both axons and the endoneurium. Fibrosis within the endoneurium prevents complete axonal regeneration and recovery. Surgical intervention to release scarred tissues or areas of entrapment is indicated to optimize functional recovery. In the absence of surgery, recovery is incomplete. Typically, outcomes following decompression are superior to excision of the injured nerve and grafting, except in cases of complex regional pain syndromes. Fourth- and fifth-degree injuries are unfavorable. Fourth-degree injuries, or “neuromas-in-continuity,” include injuries to axons, endoneurium, and perineurium. No potential for spontaneous recovery exists with fourth-degree injuries, and surgical intervention with neuroma excision and grafting is required. Superior long-term outcomes with neuroma excision and grafting, rather than neurolysis have been demonstrated in the example of birth-related brachial plexus injuries. Fifth-degree nerve injuries, or “neurotmesis,” result from complete nerve transection, or interruption of axons and all connective tissues (endoneurium, perineurium, and epineurium). Surgical management is required. Sixth-degree, or “mixed pattern injuries” describe nerve injuries that incorporate two or more injury patterns at the same level. These injury types are the most complex, as consideration must be given to all involved injury types with particular emphasis to not downgrade function.
The determination of nerve injury is often aided with electrodiagnostic studies. The second author (ASW) employs electromyography (EMG) to augment the clinical picture in patients with unclear degrees of injury. Fibrillations, which are indicative of nerve injury, appear within 4–6 weeks of injury, but motor unit potentials (MUPs), which result from adjacent axonal sprouting predict the capacity for spontaneous recovery, may not appear on EMG testing until 8–12 weeks after injury. EMG testing can, therefore, be employed 3 months from the time of injury to determine prognosis for spontaneous recovery. The absence of MUPs is an indication to proceed with surgical intervention. Motor unit potentials are not seen in fourth- and fifth-degree injuries, and may or may not be seen in third-degree injuries ( Table 24.1 ). EMG testing can be particularly helpful for determining prognosis in third-degree and mixed injuries.
Wallerian Degeneration and Neuroregeneration
Changes occur in both the proximal and distal neuron after injury. Dr Augustus Waller first described the “ alterations which take place in the elementary fibres of the nerve after they have been removed from their connection with the brain or spinal marrow ” in sections of the glossopharyngeal and hypoglossal nerves of the frog. Termed “Wallerian degeneration,” the distal axonal segment undergoes degenerative changes in which SCs, fibroblasts, myocytes, and injured axons express neurotrophic factors in response to injury as degrading neural elements are phagocytosed. Axons in the proximal segment undergo retraction and a brief dormant phase before formation of a regenerating unit. The regenerating unit elongates and sprouts multiple axon branches. Remaining endoneurial tubes termed “Bands of Bungner” and proregenerative Schwann cells guide axons to their targets. Extreme proximal axonal injuries and avulsions may lead to death of the cell body. Similar processes of Wallerian degeneration and neuroregeneration occur in the setting of nerve autografting and are required for proper regeneration across the graft. Increased knowledge of the molecular signaling events occurring during these degenerative and regenerative processes may lead to therapeutic interventions for management of neurodegenerative disorders.
Planning the Reconstruction
Timing of Nerve Repair/Reconstruction
Recovery following peripheral nerve injury is influenced not only by the degree of nerve injury, but also by the relative distance of the injury to the end-target. When planning motor reconstruction, consideration must be given to the timing of the nerve injury, timing and type of nerve repair or reconstruction, and required nerve regeneration distance for reinnervation of the target muscle. There is a critical window of approximately 12–18 months, during which functional reinnervation is possible following injury. Beyond this timeframe, muscle and nerve are unable to interface for a multitude of reasons.
After nerve injury, neurons, Schwann cells, and muscle all undergo changes in morphology and genetic expression to prepare and allow for regeneration to occur. Previously, muscle was thought to be the primary contributor to poor recovery with prolonged denervation. Myofibril reabsorption, actin and myosin catabolism, increased collagen deposition in the extracellular space, and decreased muscle cell size occur due to proteasomes associated with the ubiquitin-proteasome pathway. Myocytes may undergo apoptosis, and muscle becomes less vascular due to capillary loss. These changes occur non-uniformly throughout muscle. Muscle fibers group with reinnervation, and although motor endplate number may increase in the remaining viable muscle during reinnervation, functional recovery is poor. Loss of muscle cross-sectional area in denervation atrophy corresponds with decreased contractile force. With prolonged denervation, myofibril disorganization and collagen replacement result in decreased specific force capacity, which is permanent. Axons distal to the injury undergo fragmentation and Wallerian degeneration, with loss of their myelin sheath, as outlined above. Denervated SCs dedifferentiate to a less mature state that supports neuroregeneration and neuromuscular junction reinnervation. Prolonged denervation and axotomy periods, however, lead to progressively poor neuroregenerative conditions involving not only the muscle, but also the nerve. There is increasing evidence regarding the deleterious effect of SC senescence on neuroregeneration. The complete microenvironment, therefore, becomes less supportive for functional recovery with prolonged denervation.
It is this time-sensitivity for motor recovery that is integral for reconstructive planning. The time interval from injury to presentation to the peripheral nerve surgeon, as well as the location of the nerve lesion and its distance from the motor end-target, may preclude optimal or even feasible functional motor recovery via traditional methods. Proximal nerve injuries that are distant from their end-target may not be appropriate for reconstruction at the site of the lesion, even with immediate patient presentation. Nerve transfers provide a solution to this reconstructive challenge by reconstructing the deficit near the end-target rather than at a distant injury site. Nerve transfers are discussed further in a later section.
Sensory reinnervation is not limited by such time constraints, although the unfavorable microenvironment related to axons and SCs continues to be investigated. Currently, it is accepted that sensory reconstruction may be performed at any time and may traverse any distance, although the true boundaries of sensory reconstruction remain unknown.
Sensory Protection of Denervated Muscle
Given that time to motor reinnervation is inversely related to functional outcome, opportunities to provide protection for denervated muscle during the neuroregeneration period have been pursued. Protection of denervated muscle via sensory innervation has been described. Temporary or permanent sensory protection has demonstrated increased muscle mass, increased compound motor action potentials, and decreased levels of glial cell line-derived neurotrophic factor mRNA compared with unprotected controls, suggesting a trophic effect for denervated muscle fibers. Sensory-protected muscles demonstrate retained muscle fiber organization, a trend in increased motor endplate numbers, and reduction in muscle spindle degeneration. The functional benefits for sensory protection of denervated muscle remain uncertain, but clinical improvements have been reported by Bain et al. after saphenous nerve protection of the tibialis anterior branch of the peroneal nerve and the gastrocnemius branch of the tibial nerve in the setting of complete sciatic nerve palsy following total hip arthroplasty.
Principles of Nerve Repair
Equipment
Peripheral nerve surgery should be performed by an experienced or well-trained microsurgeon familiar with peripheral nerve anatomy and physiology, with the proper available equipment, team, and facilities. Nerve surgery is best performed with the use of the operating microscope with appropriate lighting, although some surgeons prefer the use of ≥4.0 loupe magnification. The neurotomy instrument set (S&T, USA) greatly facilitates cutting the nerve sharply ( Fig. 24.2 ) to maintain all fascicles in the same plane. We prefer 10-0 nylon with an atraumatic needle for perineurial suture and 10-0–8-0 nylon for epineurial sutures. Larger suture materials are not used, even when major nerves are being repaired. Suture materials for nerve repair allow approximation and alignment of the nerve ends, not strength to withstand tension. All nerve coaptations should be tension-free. Sharp-tip needles are imperative, and each needle should be used for only four or five stitches, since multiple passes through the nerve blunts the tip of the needle. Dull needle tips may dislodge delicate fascicles and prevent atraumatic needle passage. Fibrin may be used as a safe alternative to microsutures for neural coaptations, such as in birth-related brachial plexus surgery, where proximal stumps may be located within spinal foramina, or as reinforcement for a sutured neural coaptation.

Operative Considerations
Neurorrhaphy Techniques
Nerve repair and reconstruction must be performed with a healthy nerve. Healthy nerves are supple with discernible fascicles that tend to gently pout, or minimally protrude, from a sharply cut end. Elegant nerve reconstructions performed within the zone of nerve injury have no functional value. In the case of an acute, sharp nerve transection, surgical debridement of the transected nerve ends is probably unnecessary. However, in cases of nerve avulsion, crush, blast, or mixed patterns of injury, neural debridement is required. Demarcation of injured nerve ends is complete 3 weeks after injury. Careful dissection is required to identify the nerve itself. Electrical stimulation should always be utilized intraoperatively to augment dissection and confirm diagnosis. Transected nerves stimulate for 72 h after injury before neurotransmitters are exhausted. Beyond 72 h after nerve transection, nerve stimulation will no longer be possible. Neural debridement begins with division at the most scarred portion of the neuroma or transected stumps and progresses in a step-wise, cut-by-cut fashion, which is termed “breadloafing” (Dr Susan Mackinnon, pers. comm.), until a healthy fascicular pattern is observed. Frozen sections may be performed intraoperatively to verify absence of fibrosis before proceeding with nerve repair.
All neural coaptations must be performed free of tension. This technical detail is imperative to successful outcomes. Excessive tension prevents neuroregeneration due to impaired microvascular flow through the vasa nervorum at the endoneurium and due to creation of increased scarring. Adjacent joints should never be flexed to allow primary nerve coaptation. Ideally, nerves should have a lazy “s” pattern at coaptation sites ( Fig. 24.3 ), and joints should be fully ranged to ensure no tension with changes in position. This technique not only ensures optimal regenerative conditions with vascularity, but also minimizes the need for postoperative splinting and therefore allows faster initiation of nerve gliding with movement to minimize scar formation. If this is not possible, a nerve graft or some other nerve repair technique should be employed. Similarly, sutures should not be pulled taut, but instead should be sufficient to just approximate nerve stumps without bending the fascicular ends, which impedes regeneration.

Whenever possible, proper fascicular orientation should be performed for coaptation of the motor and sensory fascicles between each nerve stump. In acute cases (<3 days after injury), the distal motor nerve can be distinguished by electric stimulation. Orientation of the proximal fascicles, however, requires electrophysiologic or biochemical examination. We do not routinely apply this time-consuming method, and rely on the orientation of the epineurial vessels for proper alignment.
Historically, controversy exists between proponents of the epineurial and the perineurial suture, but both techniques and their modifications may be utilized in the clinical setting. Epineurial suturing may be appropriate, for example, to repair a sharp laceration of the median nerve at the wrist, while intercostal nerve transfers require a perineurial suture technique. For all techniques, any stray or escaping fascicles should be trimmed to allow straight apposition of fascicular ends. In this section, each technique is described in detail.
Epineurial Suture
Following orientation of the fascicles, the epineurial suture commences with two laterally placed 8-0 or 9-0 nylon sutures, passing the needle from the epineurium to the internal epineurium ( Fig. 24.4A ), tied to align the fascicles between each nerve stump, taking care not to cinch sutures tightly. Interrupted sutures, as necessary, are added between the first two stay sutures on the anterior aspect. The nerve is then rotated by manipulation of the lateral stay sutures, so that the posterior epineurium can be gently approximated. Tight sutures tend to twist the fascicles within the epineurium ( Fig. 24.5A ), which can be prevented by the assistant applying mild traction to both sites of the nerve ( Fig. 24.5B ). This technique is useful to suture the nerve in a fresh wound or for nerve transfer, where the epineurium is thin and retracted.


Perineurial Suture
For perineurial coaptations, the mesoneurium and external and internal epineurium are removed from both ends of the nerve stump with microsurgery scissors. Following orientation and matching of fascicles between both stumps, each fascicle is coapted with 10-0 nylon by passing the needle through the perineurium close to the edge, using a small bite so as not to slip off both ends of the fascicles ( Fig. 24.4B ). The assistant surgeon can hold both ends of the nerve under mild tension to facilitate this maneuver. The repair of each fascicle is completed with three or four sutures of 10-0 nylon. All matched fascicles are connected in the same fashion.
Epiperineurial Combined Suture
The senior author (KD) prefers epiperineurial combined sutures even when the conventional epineurial suture is indicated because this allows more accurate coaptation of the peripheral fascicles within the epineurium. Nylon sutures (8-0 or 9-0) are passed through the epineurium and perineurium simultaneously with the same pass of the needle, and tied ( Fig. 24.4C ). In this technique, the lateral stay sutures are not applied because the remaining fascicles cannot be seen after the two lateral sutures are placed. Suturing is performed in a circumferential order, taking care to coapt the remaining fascicles as much as possible ( Fig. 24.6 ).

Nerve Reconstruction
Nerve repair and reconstruction in the 21st century may incorporate multiple techniques. The goals of PNS reconstruction are to maximize functional restoration safely and efficiently, while minimizing any donor deficit. The mechanism and degree of injury; location of lesion; age of patient; associated injuries including life-threatening or systemic disease or injury, adjacent vascular injuries or complex soft tissue wounds; the time since the injury occurred; and the availability of donor tissues are considered to determine the optimal reconstruction for an individual patient. Specific treatment algorithms have been previously reviewed. Primary nerve repair, interpositional nerve grafting, grafting with nerve substitutes, nerve transfers, and nerve allotransplantation may be used. Nerve allotransplantation and nerve substitutes are covered thoroughly in Chapter 68 and therefore are not discussed here.
Primary Nerve Repair
Direct repair of transected ends is the repair of choice when appropriate conditions exist. Primary repair is typically performed after sharp transection (fifth-degree, unfavorable) injuries. Repair is ideally performed within 72 h of injury to facilitate the use of electrical stimulation for identification of distal nerve stumps. A simple, interrupted microsuturing technique is used to reapproximate nerve ends ( Fig. 24.4A ).
Nerve Grafts
Autogenous nerve grafts are the gold standard reconstruction for nerve gaps. Nerve autografts provide both a matrix and viable SCs to support nerve regeneration. When planning a nerve repair, the length of the nerve gap, the diameter of the injured nerve, the fascicular pattern of both ends of the nerve stumps, and the vascularity of the surrounding recipient bed are taken into consideration for selection of the donor nerve and its length. We most frequently utilize the sural nerve as a donor autograft due to the ease of harvest and minimal donor morbidity, but other frequently used donor autografts and their characteristics are reviewed in Chapter 68 ( Table 68.2 ). The median and lateral antebrachial cutaneous (MABC and LABC) nerves serve as excellent donors for upper extremity reconstruction. Motor nerves are ideally grafted with motor autografts for improved regeneration, due to preferential motor regeneration, or the tendency for regenerating motor axons to prefer motor pathways over sensory pathways when given the choice, but available motor donors are typically quite limited. Kawamura et al. demonstrated that motor, sensory, and mixed nerve grafts result in equivalent regeneration for purely motor or purely sensory unifascicular nerves and conclude that the advantage of motor grafts exists in the setting of regeneration of mixed motor and sensory nerves due to the increased space in motor grafts for motor and sensory regeneration competition to occur. Depending on the discrepancy in diameter between the recipient and donor nerves, the number of necessary nerve cables should be calculated. We usually assume a 20% longer nerve graft than the actual length of gap to prevent tension after nerve grafting. All nerve repairs should be performed in a tension-free manner, and nerve grafts should have the same lazy “s” configuration as any coaptations ( Fig. 24.3 ).
Nerve grafts may include standard interpositional autografting, interfascicular autografting, or vascularized nerve autografting. Endoscopic techniques are frequently employed for sural nerve graft harvest. Vascularized nerve grafts (VNG) are used less frequently clinically, but may be beneficial for a long-segment nerve defect, large diameter nerve, or a severely scarred recipient bed, which obstructs neovascularization of the nerve graft. Small diameter nerve grafts spontaneously revascularize, but larger caliber nerves do not revascularize as readily, and therefore may benefit from a VNG. Nerve grafts in severely scarred tissues may benefit from replacement of the surrounding tissue by a vascularized (free or pedicled) fasciocutaneous flap or VNG, even for a short nerve gap. The vascularized sural nerve and ulnar nerve grafts are well-described ( Figs 24.7 , 24.8 ). Superior regeneration has been reported in a VNG compared with non-vascularized nerve autograft in a single patient case study. Terzis and Kostopoulos reported their experience with 14 VNGs in lower extremity reconstruction and noted the benefits of a VNG for long defects (>13 cm) and in traction injuries (due to the lengthy zone of injury), especially if performed within 6 months after the injury. Still, VNG are not frequently used, possibly due to the length and complexity of the procedure, limited donors, and availability of alternative reconstructive techniques. The coaptation techniques for conventional sural nerve grafting, interfascicular nerve grafting, and vascularized nerve grafting are reviewed.


Conventional Sural Nerve Grafting
The sural nerve ( Fig. 24.9 ) is a frequent donor nerve due to its relative ease of harvest and well-tolerated donor deficit. The proximal portion of the sural nerve, just distal to the popliteal crease, is typically free of branches. A nerve stripper device can be utilized to facilitate harvest of the nerve, and multiple types of incisions may be employed. The caliber of the nerve increases distally near the ankle, and this knowledge may be advantageous for improving the size match of the coaptation, depending on the recipient nerve. The surrounding mesoneurium and fatty tissue around the harvested sural nerve are removed with microsurgery scissors under the operating microscope ( Fig. 24.10A ) and placed between both ends of the recipient nerve. Depending on the length of nerve gap and diameter of the recipient nerve, the sural nerve is folded and cut into segments long enough to bridge the gap ( Fig. 24.10B ). The direction of the donor nerve is not of major concern if no branches are present. If branches are present, the nerve is reversed to prevent axonal drop-off.
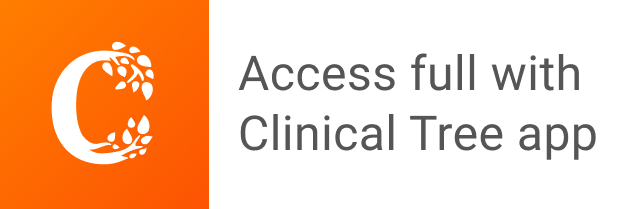