(9.1)
where C 0 is the concentration of the solute in the donor chamber, K is the partition coefficient, or log P of the solute into skin (SC), D is the effective diffusion coefficient across the skin, L is the path length of diffusion.
At steady state
, Eq. (9.1) above can be rewritten in a simpler form as
![$$ {M}_t=\frac{C_0 KD}{L}\left[ t-\frac{L^2}{6 D}\right] $$](https://i0.wp.com/plasticsurgerykey.com/wp-content/uploads/2017/07/A309387_1_En_9_Chapter_Equ2.gif?w=960)
This represents the permeability profile of a solute diffusing across skin. The slope of this profile provides flux of the solute across skin,

The permeability profile is linear in time but exhibits a lag time,

![$$ {M}_t=\frac{C_0 KD}{L}\left[ t-\frac{L^2}{6 D}\right] $$](https://i0.wp.com/plasticsurgerykey.com/wp-content/uploads/2017/07/A309387_1_En_9_Chapter_Equ2.gif?w=960)
(9.2)

(9.3)

The terms K, D and L are grouped together and defined as a single term,
, the skin permeability. Solute permeability can then be estimated from flux of the solute across the skin and its concentration in the donor chamber.

Several simplifying assumptions have been made in deriving this relationship (Foreman and Kelly 1976; Osborne 1986). Nevertheless, Eq. (9.4) above provides a straightforward way of determining skin permeability to different solutes by measuring their flux.


(9.4)
9.5.1.2 Model Solutes
The solute, whose permeability is to be assessed across the skin, needs to be detected in the receiver chamber by means of appropriate analytical methods. These may include spectrometry, chromatography, biochemical methods such as ELISA, western blots, etc. The solute itself may be labeled using a fluoropore or radioisotope for direct detection. Care needs to be taken that labeling of the solute does not alter its physicochemical properties, which may affect skin permeability resulting in misleading conclusions.
9.5.1.3 Diffusion Cells
A wide variety of diffusion cell systems have been developed for measuring solute permeation through membranes (Frantz 1990; Bronaugh and Collier 1991; Gummer and Maibach 1991; Friend 1992). The most common configurations are vertical cells, where the donor chamber is atop the receiver chamber separated by the membrane in between, and horizontal diffusion cells where the donor and receiver chamber are arranged side-by-side. Mixing of the chambers to create homogeneous compartments is critical in horizontal diffusion cells. In case of vertical diffusion cells, mixing is not critical. However, a homogeneous well-mixed receiver chamber better mimics in vivo conditions and prevents the formation of a static boundary layer of high-solute concentration in the receiver chamber. Formation of unmixed zones is especially critical when assessing the permeability of a hydrophobic solute in an aqueous receiver compartment (Tsuruta 1977; Bronaugh and Stewart 1984, 1986). Efficient mixing can be obtained using small magnetic stir bars. Flow-through diffusion cells in which the receptor fluid is continuously refreshed to mimic in vivo sink conditions (i.e., metabolism and diffusion into the subdermal vasculature) have also been used successfully (Ainsworth 1960). Temperature control of the diffusion cell can be attained using water jackets or simply submerging the entire cell assembly into a water bath. For studying permeation of highly hydrophobic compounds, solubilizing solvents can be added to the aqueous receiver chamber. These include Triton X-lOO (Bronaugh and Stewart 1984), bovine serum albumin (Brown and Ulsamer 1975), Poloxamer 188 (Hoelgaard and Mollgaard 1982), PEG 400 (Valia et al. 1984), and ethanol (Scott et al. 1986). Caution needs to be exercised when using solubilizing agents that they do not alter inherent membrane properties. Hydration effect on membrane integrity needs to be considered when assessing solute permeability over extended periods of time. Some studies have reported that long-term hydration in rodent skin, and in particular hairless mouse skin, can lead to changes in permeation rates (Whitton and Everall 1973; Bond and Barry 1988a, b, c, d; Hinz et al. 1989). Finally, the area of diffusion of the donor chamber has been shown to have some effects on the permeation rates (Karande and Mitragotri 2003). Water penetration into the skin introduces a lateral strain at the edges of the donor chamber due to swelling. This scales as the strain edge available per unit area and results in higher observed permeation rates in smaller donor chambers.
- (a)
Horizontal diffusion cells
Several designs have been suggested and used successfully for this type of diffusion cell. These include the T-shape configuration (Washitake et al. 1980), L-shape configuration (Dyer et al. 1979; Tojo et al. 1985a), glass conical flasks configuration (Wurster et al. 1979), vertical membrane, equi-compartment diffusion cell with high area to volume ratio (Flynn and Smith 1971), glass diffusion cells with steel mesh (Southwell and Barry 1983), Valia–Chien cells (Tojo et al. 1985a, b), and flow through system with central inlet and peripheral effluent ports (Astley and Levine 1976). In recent years, several modified versions of these early designs have been used (Morell et al. 1996; Aramaki et al. 2003; Bakand et al. 2006; Soni et al. 2006; Tas et al. 2007).
- (b)
Vertical cells
Vertical diffusion cells are closer to in vivo situation in obtaining permeability data across the skin (Friend 1992). The Coldman cell represents the earliest of all vertical diffusion cells (Coldman et al. 1969). A glass cell with a side arm for sampling and stir bar for mixing forms the receiver chamber. Skin is sandwiched between the donor and receiver using a clamp. Whitton et al. studied a similar cell with the sampling arm located at the bottom of the receiver (Whitton and Everall 1973). The Franz diffusion cell remains the most widely studied vertical diffusion cell today (Franz 1978). The original design had poor mixing properties which have been addressed in subsequent modifications (Nacht et al. 1981; Loftsson 1982; Kao et al. 1983; Dugard et al. 1984; Hawkins and Reifenrath 1986; Gummer et al. 1987; Tiemessen et al. 1989). In the past two decades several modifications of the Coldman cell have been used successfully. These include the release cells (Morell et al. 1996), enhancer cells (Bosman et al. 1996), Kelder cells in combination with the Automatic Sample Preparation with Extraction Columns system (Bosman et al. 1996), Oak Ridge National Laboratory Skin Permeability Chamber (Holland et al. 1984), and Ussing type chambers (Li et al. 2006; Ito et al. 2007).
- (c)
Skin Flaps
In addition to the conventional diffusion systems discussed above, novel in vitro systems that measure effect of perfusion rates on solute permeation have also been designed. Isolated Perfused Porcine Skin Flap (IPPSF) is a model system of porcine skin flap perfused by the caudal superficial epigastric artery and its associated veins and mounted on a diffusion cell (Williams et al. 1990; Riviere et al. 1991). Bovine udder is used in a similar fashion for permeation studies (Kietzmann et al. 1993).
9.5.2 Tape Stripping
Tape stripping is a technique that has been found useful in dermatopathological and dermatopharmacological research for selectively or at times exhaustively removing the SC (Surber et al. 1999). Typically, an adhesive tape is applied to the skin and removed abruptly. This application can be repeated between 10 and 100 times (Sheth et al. 1987; Ohman and Vahlquist 1994). The observation that skin may serve as a reservoir for chemicals was first reported in 1955 (Malkinson and Ferguson 1955). Drugs like corticosteroids were shown to localize within the SC (Vickers 1963; Carr and Wieland 1966). These observations led to the use of the tape stripping technique in investigating the barrier and reservoir function of the skin (Rougier et al. 1983; Tojo and Lee 1989). This technique is now being increasingly used in measuring drug concentration and its concentration profile in the SC (Pershing et al. 1990; Pellett et al. 1997; Shah et al. 1998).
9.5.2.1 Theory
Solute diffusion in the SC can be described by Fick’s law (Crank 1975) as follows

where D is the average solute diffusion coefficient in the SC, C s is the solute concentration in the SC, and x is the distance from the SC surface. Eq. (9.1) can be solved with the following boundary conditions:
where x = 0 corresponds to the SC surface and x = L corresponds to the end of the SC, K is the average solute partition coefficient in the SC, and C 0 is the donor concentration of the solute. The resulting equation for solute concentration in the SC, C s is given as follows (Crank 1975):

where C ∞ is the solute concentration in SC at steady state (
). For short times, i.e., low values of
, Eq. (9.6) can be simplified as

Using the definition of permeability P, the above Eq. (9.7) further simplifies to

Equation (9.8) shows that the solute concentration in the SC measured at short times is proportional to its steady-state permeability. Accordingly, solute concentration can be measured via tape stripping in the SC to infer its steady-state permeability. An analytical technique such as high-performance liquid chromatography (HPLC) or an immunoassay or radioimmunoassay is required in conjunction to accurately determine solute concentration. Several studies have successfully applied this method to determine the skin permeability of a wide range of solutes (Stinchcomb et al. 1999; Alberti et al. 2001a, b, c; Moser et al. 2001).

(9.5)


(9.6)



(9.7)

(9.8)
9.5.3 Impedance Spectroscopy
Methods based on measuring solute diffusion across skin may not always provide the sensitivity required to measure small perturbations in skin permeability or follow permeability changes over short intervals of time. Electrical measurements across the SC provide improved sensitivity (Dugard and Scheuple 1973). Electrical properties of SC parallel those of permeability and play a dominant role in the control of current flow (Lawler et al. 1960; Tregear 1966). A review of factors governing the passage of electricity across skin has been presented by Tregear (Tregear 1966).
9.5.3.1 Theory
Flow of ions across skin under an electric field is analogous to diffusion of solutes under a chemical gradient. Current across skin can thus be related to permeability of skin. Skin and its appendages can be represented by an equivalent circuit containing a resistance R shunted by capacitance C (Lackermeier et al. 1999). The impedance, Z, of this equivalent skin model can be represented as
![$$ Z={\left[{R}^2+\frac{1}{{\left(2\pi fC\right)}^2}\right]}^{\frac{1}{2}} $$](https://i0.wp.com/plasticsurgerykey.com/wp-content/uploads/2017/07/A309387_1_En_9_Chapter_Equ9.gif?w=960)
where f is the frequency of the applied alternating current (AC) signal. A formal porous pathway theory based on Nernst–Planck flux equations and the Nernst–Einstein relations for ideal solutions has been developed that relates skin impedance to skin permeability (Lakshminarayanaiah 1965; Srinivasan and Higuchi 1990; Li et al. 1998, 1999; Tezel et al. 2003).
![$$ Z={\left[{R}^2+\frac{1}{{\left(2\pi fC\right)}^2}\right]}^{\frac{1}{2}} $$](https://i0.wp.com/plasticsurgerykey.com/wp-content/uploads/2017/07/A309387_1_En_9_Chapter_Equ9.gif?w=960)
(9.9)
A simplified correlation between skin resistivity, R, and skin permeability, P, is provided by Tang et al. (2001) as

where C is dependent on the properties of the solute and the solvent in which it is dissolved.

(9.10)
The impedance of intact skin is in the range of several hundred kilo ohms (kΩ). As the skin is permeabilized, impedance drops finally attaining a value of ~1 kΩ, which corresponds to removal of the entire barrier (Naik et al. 2001). Skin impedance measurements have been used to study the effect of sonophoresis (Mitragotri et al. 1996; Tezel et al. 2001; Paliwal et al. 2006), iontophoresis (Burnette and Bagniefski 1988; Kalia et al. 1996; Kumar and Lin 2008), and chemical enhancers on skin permeability (Karande et al. 2004; Karande et al. 2006a, b).
In comparison to diffusion measurements of solute permeability in diffusion cells, electrical impedance measurements are relatively simpler, faster, and more sensitive. Impedance-based permeability assessment provides direct readout of barrier integrity and does not require subsequent analysis, which may be tedious, time consuming, and expensive. Also, since these measurements can be performed rapidly and by use of automated systems the throughput of impedance-based assays is significantly larger compared to diffusion cells. Karande et al. have described the design of an impedance based high-throughput assay to determine the effect of chemical permeation enhancers on skin permeability (Karande et al. 2004, 2006a, b). This system, in vitro impedance Guided High Throughput (INSIGHT) screen, is capable of assessing thousands of formulations per day for their ability to modulate skin permeability. The authors discovered synergistic formulations of permeation enhancers using this screen, which were capable of delivering a biologically active hormone across the skin at therapeutically relevant doses. One downside of using impedance to assess skin permeability is that impedance serves only as a surrogate measure of actual skin permeability. The actual flux of a solute needs to be assessed by conventional diffusion methods.
9.5.4 Infrared Spectroscopy
Transport of solute molecules in the skin can be studied using spectroscopic techniques. Fourier transform infrared (FTIR) spectroscopy has been used extensively in determining skin structure, properties, hydration, and effect of permeation enhancers (Potts and Francoeur 1993; Moore and Rerek 1998; Karande et al. 2005; Mendelsohn et al. 2006).
9.5.4.1 Theory
FTIR spectroscopy is used to track solute molecules in the epidermis by recording their molecular vibrations. The integrated absorbance of these vibrations is directly proportional to the amount of solute present in the skin. Depth-dependant profiling of the solute in the skin can be achieved by attenuated total reflectance (ATR)-FTIR spectroscopy. ATR-FTIR spectroscopy generally provides information in the superficial 1–2 μm layer of the skin. Repeated tape stripping can be used to scan successive layers of the skin for solute penetration. Transport properties for the solute can then be obtained from an unsteady state solution for Eq. (9.5) given as (Pirot et al. 1997)

where C(x) is the concentration of solute at a depth x from skin surface and C 0 is the concentration of the solute at the skin surface. L is the total thickness of skin and D is the effective diffusivity of the solute in skin. The rate per unit area at which the solute diffuses out of the skin can be obtained by differentiation of Eq. (9.11) as
. Further, integration of this rate with time yields the total amount of solute diffusing across skin in a given time. This information can be used to obtain the permeability profile of the solute and hence its permeability across the skin. Several assumptions have been made in deriving this correlation that is discussed in Pirot et al. (1997). One downside of using ATR-FTIR spectroscopy is that the solute to be monitored needs to be IR active and have a signature distinct from those of the SC components. This limitation can, in theory, be overcome using spectral correction techniques (Naik et al. 2001).

(9.11)

Several other methods have been used to study permeation and absorption of material into and across skin. Xiao et al. have used Raman microscopy and imaging to track penetration of phospholipids in skin (Xiao et al. 2005a, b). Williams et al. provide a critical comparison of different Raman spectroscopy techniques and their application in vivo (Williams et al. 1993). Sonavane et al. have used a combination of UV-vis spectroscopy, X-ray spectroscopy, and inductive coupled mass spectroscopy to track permeation of gold nanoparticles in the rat skin (Sonavane et al. 2008). Other groups have used photoacoustic spectroscopy for studying permeation of Carbopol 940 and transdermal gels (Christ et al. 2001; Rocha et al. 2007; Rossi et al. 2008). Remittance spectroscopy and photothermal deflection are a few of the other methods that have been suggested in this area (Gotter et al. 2008).
9.5.5 Trans Epidermal Water Loss
Mammalian skin has evolved to regulate the transport of material into and out of the body. One of the primary functions of skin is to regulate the loss of water from the body. It follows, then, that the quantitative measure of water loss from the skin is indicative of its barrier integrity. A device, such as an evaporimeter, that can adequately and accurately measure the water vapor flux at the skin surface, and hence the rate of trans-epidermal water loss (TEWL), can be used to assess barrier integrity of the skin. A quantitative correlation between TEWL and skin permeability has been reported (Rougier et al. 1989) and is found to be consistently reproducible in vivo and in vitro (Pinnagoda et al. 1989, 1990). Several studies have reported the use of TEWL to assess the effect of permeation enhancers on skin permeability (Loden 1992; Kanikkannan and Singh 2002; Luzardo-Alvarez et al. 2003; Tokudome and Sugibayashi 2004). A comprehensive review of their findings is provided by Levin and Maibach (Levin and Maibach 2005). This review also sheds light on possible reasons why two particular studies did not observe quantitative correlation between percutaneous absorption and TEWL (Tsai et al. 2001; Chilcott et al. 2002). Recent literature continues to provide opposing views on the use of TEWL as a measure of skin barrier integrity (Fluhr et al. 2006; Netzlaff et al. 2006a). Nevertheless, several commercial devices based on this principle are readily available today for laboratory work.
9.6 Evaluation of Skin Permeability In Vivo
In vivo methods for quantification of solute permeation across the skin serve as a gold standard in transdermal drug delivery. Such methods can potentially eliminate variables associated with using excised human or animal skin, surrogate endpoints as well as faithfully reproduce metabolic, pharmacokinetic, and pharmacodynamic behavior of the drug molecule. Wherever possible, and practically feasible, in vivo measurements are considered reliable and superior to measurements made on model systems in vitro.
9.6.1 Diffusion Measurements
Diffusion cells have been designed that can be filled with drug solution and strapped on to an animal in vivo or the arm of a human volunteer (Wurster and Kramer 1961; Quisno and Doyle 1983; Leopold and Lippold 1992). Such a system allows one to study the passive permeation of a solute across the skin in vivo in much the same way as diffusion cells with excised skin or model membranes. Different endpoints can then be used to quantify permeation rates across the skin.
9.6.1.1 Systemic Bioavailability
A solute whose percutaneous absorption is to be measured is applied topically to the skin and its concentration measured in blood plasma or urine over a period of time. These amounts generally tend to be very low and hence a sensitive detection technique such as radiolabeling with 3H or 14C is necessary (Wester et al. 1983; Gschwind et al. 2008). Caution needs to be exercised when using such techniques if the drug is susceptible to metabolism. Metabolism can lead to by-products that have significantly different pharmacokinetics and pharmacodynamics than the parent compound and can give misleading results.
9.6.1.2 Surface Loss
Alternate approach to determine in vivo percutaneous absorption is to measure the loss of solute from the surface as it penetrates the skin. This can be achieved when all residual material, contained in a reservoir, can be recovered. Difference between concentration or amount at time zero and at time of recovery provides an estimate of amount absorbed. Generally, the method used to detect the residual amount needs to be sensitive enough, especially in supersaturated systems, as the reservoir can be infinitely large when compared to the amount absorbed. Also, this method assumes that the skin does not act as a reservoir, which in itself can be a poor assumption.
9.6.2 Pharmacological Response
A good method to assess percutaneous absorption is to measure the biological or pharmacological response to the drug. Vasoconstriction can be used as an endpoint to measure transdermal delivery of topically applied corticosteroids (Barry 1983) and vasodilation as an endpoint for topically applied nicotinates (Le and Lippold 1995). Laser Doppler flowmetry (LDF) has been used to study absorption of prostaglandin E1 (PGE1) (Foldvari et al. 1998), methylnicotinate (Wilkin et al. 1985; Poelman et al. 1989), and minoxidil (Wester et al. 1984) in vivo by measuring changes in cutaneous microcirculation. Reduction in blood glucose levels has been used as an endpoint to determine the efficacy of transdermal delivery of insulin (Mitragotri et al. 1995; Chen et al. 2006). The downside of this method is that it works only for drugs that have a detectable biological endpoint and can tend to be more qualitative than quantitative.
9.6.3 Other Approaches
Several of the methods discussed above for in vitro skin barrier assessment can be adopted for in vivo measurements with little or no modification at all. Tape stripping and TEWL measurements in particular are attractive for in vivo measurements as they are relatively straightforward and minimally invasive or noninvasive. The amount of solute that penetrates the SC can be quantified in vivo, in humans, by tape stripping with an appropriate adhesive tape (Tsai et al. 1991). In case of animal studies, in vivo, it is possible to quantify penetration in deeper skin layers by sacrificing the animal and harvesting its skin. The concentration profiles can then be used to determine solute permeability as suggested in Eq. (9.8). Umemura et al. have used tape stripping in vivo in healthy human subjects to determine the pharmacokinetics of topically applied maxacalcitol from an ointment and lotion (Umemura et al. 2008). Puglia et al. have used tape stripping to quantify skin penetration of lipid nanoparticles (Puglia et al. 2008). A review of tape-stripping methods in determining percutaneous absorption in vivo is provided by Herkenne et al. (Herkenne et al. 2008). Several reports have documented the use of TEWL to assess the barrier integrity after treatment with physical or chemical enhancers of skin permeability (Atrux-Tallau et al. 2008; Kolli and Banga 2008). Maibach et al. have documented the effect of successive tape strippings on TEWL in vivo in human subjects as well as compared two different configurations for devices measuring TEWL (Zhai et al. 2007). Xhauflaire-Uhoda et al. have used TEWL measurements to study barrier repair after the application of miconazole nitrate and tape stripping (Xhauflaire-Uhoda et al. 2006). Among the various spectroscopic techniques, ATR-FTIR spectroscopy has been used extensively in tracking solute permeation in the skin (Ayala-Bravo et al. 2003; Tsai et al. 2003; Curdy et al. 2004; Escobar-Chavez et al. 2005; Remane et al. 2006). A comprehensive review of ATR-FTIR spectroscopy and its use in in vivo studies appears in Naik et al. (Naik et al. 2001). Raman spectroscopy, similar in principle to FTIR spectroscopy, has also been used in studying transdermal solute penetration in vivo. A big advantage of Raman spectroscopy is that it does not require tape stripping of the skin for depth profiling and is thus a truly noninvasive technique. Stamatas et al. have used in vivo confocal Raman microspectroscopy to study the uptake of vegetable oils and paraffin oil in infants (Stamatas et al. 2008). Pudney et al. have demonstrated the use of Raman spectroscopy to obtain depth profiles of trans-retinol in the epidermis for 10 h after application in an in vivo setting (Pudney et al. 2007). Caspers et al. have used confocal Raman spectroscopy to detect dimethyl sulfoxide in the SC (Caspers et al. 2002). For both techniques, ATR-FTIR and Raman spectroscopy, the molecule of interest should have an active IR signature of sufficient intensity that is distinct from the signatures of skin components. An additional drawback of using Raman spectroscopy is that it can only provide relative concentrations as against absolute amounts of the diffusing solute in different layers of the skin. Impedance spectroscopy is a highly sensitive and relatively straightforward technique that can be used in vivo to assess skin barrier integrity. Curdy et al. have used impedance spectroscopy to follow barrier recovery after the application of iontophoresis (Curdy et al. 2002). Dujardin et al. describe the use of impedance measurements to assess effects of electroporation on barrier function in vivo in rats (Dujardin et al. 2002). Kalia et al. have looked at the effect of surfactant treatment and iontophoresis on skin impedance in vivo (Kalia and Guy 1997).
In addition, several other techniques have been utilized to quantify in vivo transdermal delivery (Herkenne et al. 2008). These include creation of suction blisters and punch biopsies. Although relatively straightforward, these are painful and invasive procedures that are less popular in studying percutaneous absorption of solutes, especially in human subjects. Recently, microdialysis has been suggested as a novel technique to measure the diffusion of solutes across the skin (Kreilgaard 2002; Mathy et al. 2005). A thin probe perfused with a physiological solution is implanted under the dermis where, on equilibration, it can exchange material with the extracellular tissue components by passive diffusion. The perfusate from the probe can then be analyzed for the solute diffusing across the skin. A time-based concentration profile of the solute diffusing across the skin into the probe can be used to determine the pharmacokinetics of the solute. A practical challenge associated with this method is the careful and reproducible insertion of the probe in the deeper layers of the skin. Also, extremely sensitive detection methods are required to analyze the small amounts of material obtained from the perfusate.
Conclusion
The field of transdermal drug delivery research has come of age and is rich with opportunities and promises. A combination of improved representative systems that meet regulatory considerations and capture relevant biophysical properties of the skin, reliable and accurate quantification methods, as well as innovative skin permeabilization strategies will expedite the appearance of transdermal delivery systems in this new century.
References
Abraham W, Downing DT (1989) Permeability studies on model membranes prepared from stratum-corneum lipids. J Invest Dermatol 92(3):393
Abraham W, Wertz PW et al (1985) Linoleate-rich acylglucosylceramides of pig epidermis: structure determination by proton magnetic resonance. J Lipid Res 26:761–766PubMed
Ainsworth M (1960) Methods for measuring percutaneous absorption. J Soc Cosmet Chem 11:69–78
Akomeah FK, Martin GP et al (2007) Variability in human skin permeability in vitro: comparing penetrants with different physicochemical properties. J Pharm Sci 96(4):824–834PubMed
Alberti I, Kalia YN et al (2001a) Effect of ethanol and isopropyl myristate on the availability of topical terbinafine in human stratum corneum, in vivo. Int J Pharm 219(1–2):11–19PubMed
Alberti I, Kalia YN et al (2001b) In vivo assessment of enhanced topical delivery of terbinafine to human stratum corneum. J Control Release 71(3):319–327PubMed
Alberti I, Kalia YN et al (2001c) Assessment and prediction of the cutaneous bioavailability of topical terbinafine, in vivo, in man. Pharm Res 18(10):1472–1475PubMed
Aramaki Y, Arima H et al (2003) Intradermal delivery of antisense oligonucleotides by the pulse depolarization iontophoretic system. Biol Pharm Bull 26(10):1461–1466PubMed
Artusi M, Nicoli S et al (2004) Effect of chemical enhancers and iontophoresis on thiocolchicoside permeation across rabbit and human skin in vitro. J Pharm Sci 93(10):2431–2438PubMed
Astley JP, Levine M (1976) Effect of dimethyl-sulfoxide on permeability of human skin in vitro. J Pharm Sci 65(2):210–215PubMed
Atrux-Tallau N, Huynh NTT et al (2008) Effects of physical and chemical treatments upon biophysical properties and micro-relief of human skin. Arch Dermatol Res 300(5):243–251PubMed
Audus KL, Bartel RL et al (1990) The use of cultured epithelial and endothelial-cells for drug transport and metabolism studies. Pharm Res 7(5):435–451PubMed
Ayala-Bravo HA, Quintanar-Guerrero D et al (2003) Effects of sucrose oleate and sucrose laureate on in vivo human stratum corneum permeability. Pharm Res 20(8):1267–1273PubMed
Bach M, Lippold BC (1998) Percutaneous penetration enhancement and its quantification. Eur J Pharm Biopharm 46(1):1–13PubMed
Baden HP, Pathak MA (1967) The metabolism and function of urocanic acid in skin. J Invest Dermatol 48:11–17PubMed
Baden HP, Goldsmith EL et al (1973) A comparative study of the physiochemical properties of human keratinized tissues. Biochim Biophys Acta 322:269–278PubMed
Bakand S, Winder C et al (2006) An experimental in vitro model for dynamic direct exposure of human cells to airborne contaminants. Toxicol Lett 165(1):1–10PubMed
Balsari AL, Morelli D et al (1994) Protection against doxorubicin-induced alopecia in rats by liposome-entrapped monoclonal-antibodies. FASEB J 8(2):226–230PubMed
Barker CL, McHale MT et al (2004) The development and characterization of an in vitro model of psoriasis. J Invest Dermatol 123(5):892–901PubMed
Barry BW (1983) Dermatological formulations Percutaneous absorption. Marcel Dekker, New York
Barry BW (1988) Action of skin penetration enhancers – the lipid protein partitioning theory. Int J Cosmet Sci 10(6):281–293PubMed
Barry BW (1991) Lipid-protein-partitioning theory of skin penetration enhancement. J Control Release 15(3):237–248
Barry BW (2001a) Is transdermal drug delivery research still important today? Drug Discov Today 6(19):967–971PubMed
Barry BW (2001b) Novel mechanisms and devices to enable successful transdermal drug delivery. Eur J Pharm Sci 14(2):101–114PubMed
Barry BW (2004) Breaching the skin’s barrier to drugs. Nat Biotechnol 22(2):165–167PubMed
Barry BW, Brace AR (1977) Permeation of estrone, estradiol, estriol and dexamethasone across cellulose-acetate membrane. J Pharm Pharmacol 29(7):397–400PubMed
Barry BW, Eleini DID (1976) Influence of nonionic surfactants on permeation of hydrocortisone, dexamethasone, testosterone and progesterone across cellulose-acetate membrane. J Pharm Pharmacol 28(3):219–227PubMed
Beare JM, Cheeseman EA et al (1958) The pH of the skin surface of children with seborrheic dermatitis compared with unaffected children. Br J Dermatol 70:233PubMed
Beastall J, Guy RH et al (1986) The influence of urea on percutaneous-absorption. Pharm Res 3(5):294–297PubMed
Bell E, Ehrlich HP et al (1981) Living tissue formed in vitro and accepted as skin-equivalent tissue of full thickness. Science 211(4486):1052–1054PubMed
Ben-Shabat S, Baruch N et al (2007) Conjugates of unsaturated fatty acids with propylene glycol as potentially less-irritant skin penetration enhancers. Drug Dev Ind Pharm 33(11):1169–1175PubMed
Berenson GS, Burch GE (1951) Studies of diffusion of water through dead human skin – the effect of different environmental states and of chemical alterations of the epidermis. Am J Trop Med 31(6):842–853
Bhandari KH, Lee DX et al (2008) Evaluation of skin permeation and accumulation profiles of a highly lipophilic fatty ester. Arch Pharm Res 31(2):242–249PubMed
Blank IH (1939) Measurement of pH of the skin surface. J Invest Dermatol 2:67
Blank IH (1952) Factors which influence the water content of stratum corneum. J Invest Dermatol 18:433–440PubMed
Blank IH, Scheuplein RJ (1973) Mechanism of percutaneous absorption. IV. Penetration of nonelectrolytes (alcohols) from aqueous solutions and from pure liquids. J Invest Dermatol 60(5):286–296PubMed
Bond JR, Barry BW (1988a) Damaging effect of acetone on the permeability barrier of hairless mouse skin compared with that of human-skin. Int J Pharm 41(1–2):91–93
Bond JR, Barry BW (1988b) Hairless mouse skin is limited as a model for assessing the effects of penetration enhancers in human-skin. J Invest Dermatol 90(6):810–813PubMed
Bond JR, Barry BW (1988c) Limitations of hairless mouse skin as a model for in vitro permeation studies through human-skin – hydration damage. J Invest Dermatol 90(4):486–489PubMed
Bonina FP, Montenegro L et al (1993) In-vitro percutaneous-absorption evaluation of phenobarbital through hairless mouse, adult and premature human skin. Int J Pharm 98(1–3):93–99
Borgia SL, Schupp P et al (2008) In vitro skin absorption and drug release – a comparison of six commercial prednicarbate preparations for topical use. Eur J Pharm Biopharm 68(2):380–389
Bos JD, Meinardi MHM (2000) The 500 dalton rule for the skin penetration of chemical compounds and drugs. Exp Dermatol 9(3):165–169PubMed
Bosman IJ, Lawant AL et al (1996) Novel diffusion cell for in vitro transdermal permeation, compatible with automated dynamic sampling. J Pharm Biomed Anal 14(8–10):1015–1023PubMed
Botham PA (2004) The validation of in vitro methods for skin irritation. Toxicol Lett 149(1–3):387–390PubMed
Bouwstra JA (1997) The skin, a well organized membrane. Colloids Surf A 123–124:403–413
Bouwstra JA, Gooris GS et al (1998) Role of ceramide 1 in the molecular organisation of the stratum corneum lipids. J Lipid Res 39:186–196PubMed
Boyce ST, Christianson DJ et al (1988) Structure of a collagen-gag dermal skin substitute optimized for cultured human epidermal-keratinocytes. J Biomed Mater Res 22(10):939–957PubMed
Breathnach AS, Goodman T et al (1973) Freeze fracture replication of cells of stratum corneum of human epidermis. J Anat 114:65–81PubMedPubMedCentral
Breau LM, McGrath PJ et al (2001) Facial expression of children receiving immunizations: a principal components analysis of the child facial coding system. Clin J Pain 17(2):178–186PubMed
Bronaugh RL, Collier S (1991) Preparation of human and animal skin. In: Bronaugh RL, Maibach HI (eds) In vitro percutaneous absorption: principles, fundamentals, and applications. CRC Press, Boca Raton, pp 1–6
Bronaugh RL, Stewart RF (1984) Methods for in vitro percutaneous-absorption studies. 3. Hydrophobic compounds. J Pharm Sci 73(9):1255–1258PubMed
Bronaugh RL, Stewart RF (1986) Methods for in vitro percutaneous-absorption studies. 6. Preparation of the barrier layer. J Pharm Sci 75(5):487–491PubMed
Bronaugh RL, Stewart RF et al (1982) Methods for in vitro percutaneous absorption studies II. Animal models for human skin. Toxicol Appl Pharmacol 62(3):481–488PubMed
Bronaugh RL, Stewart RF et al (1983) Differences in permeability of rat skin related to sex and body site. J Soc Cosmet Chem 34(3):127–135
Bronaugh RL, Stewart RF et al (1986) Methods for in vitro percutaneous-absorption studies. 7. Use of excised human-skin. J Pharm Sci 75(11):1094–1097PubMed
Brown DWC, Ulsamer AG (1975) Percutaneous penetration of hexachlorophene as related to receptor solutions. Food Cosmet Toxicol 13(1):81–86PubMed
Burnette RR, Bagniefski TM (1988) Influence of constant current iontophoresis on the impedance and passive na + permeability of excised nude-mouse skin. J Pharm Sci 77(6):492–497PubMed
Carr RD, Wieland RG (1966) Corticosteroid reservoir in stratum corneum. Arch Dermatol 94(1):81–84PubMed
Caspers PJ, Williams AC et al (2002) Monitoring the penetration enhancer dimethyl sulfoxide in human stratum corneum in vivo by confocal Raman spectroscopy. Pharm Res 19(10):1577–1580PubMed
Chen H, Langer R (1998) Oral particulate delivery: status and future trends. Adv Drug Deliv Rev 34(2–3):339–350PubMed
Chen YP, Shen YY et al (2006) Transdermal protein delivery by a coadministered peptide identified via phage display. Nat Biotechnol 24(4):455–460PubMed
Chilcott RP, Dalton CH et al (2002) Transepidermal water loss does not correlate with skin barrier function in vitro. J Invest Dermatol 118(5):871–875PubMed
Cho CW, Choi JS et al (2008) Development of the ambroxol gels for enhanced transdermal delivery. Drug Dev Ind Pharm 34(3):330–335PubMed
Christ A, Szurkowski J et al (2001) Drug penetration into a membrane investigated by photoacoustic and FTIR-ATR spectroscopy. Anal Sci 17:S371–S373
Coldman MF, Poulsen BJ et al (1969) Enhancement of percutaneous absorption by use of volatile – nonvolatile systems as vehicles. J Pharm Sci 58(9):1098–1102PubMed
Cooper ER (1982) Effect of decylmethyl sulfoxide on skin penetration. In: Mittal KL, Fendler EJ (eds) Solution behaviour of surfactants: theoretical and applied aspects. Plenum Press, New York, pp 1505–1516
Cornwell PA, Barry BW (1995) Effects of penetration enhancer treatment on the statistical distribution of human skin permeabilities. Int J Pharm 117(1):101–112
Corrigan OI, Farvar MA et al (1980) Drug membrane-transport enhancement using high-energy drug polyvinylpyrrolidone (PVP) co-precipitates. Int J Pharm 5(3):229–238
Coutelegros A, Maitani Y et al (1992) Combined effects of ph, cosolvent and penetration enhancers on the in vitro buccal absorption of propranolol through excised hamster-cheek pouch. Int J Pharm 84(2):117–128
Crank J (1975) The Mathematics of diffusion. Oxford University Press, Inc., New York
Cronin MTD, Dearden JC et al (1998) An investigation of the mechanism of flux across polydimethylsiloxane membranes by use of quantitative structure-permeability relationships. J Pharm Pharmacol 50(2):143–152PubMed
Crooke RM, Crooke ST et al (1996) Effect of antisense oligonucleotides on cytokine release from human keratinocytes in an in vitro model of skin. Toxicol Appl Pharmacol 140(1):85–93PubMed
Cross SE, Pugh WJ et al (2001) Probing the effect of vehicles on topical delivery: understanding the basic relationship between solvent and solute penetration using silicone membranes. Pharm Res 18(7):999–1005PubMed
Curdy C, Kalia YN et al (2002) Post-iontophoresis recovery of human skin impedance in vivo. Eur J Pharm Biopharm 53(1):15–21PubMed
Curdy C, Naik A et al (2004) Non-invasive assessment of the effect of formulation excipients on stratum corneum barrier function in vivo. Int J Pharm 271(1–2):251–256PubMed
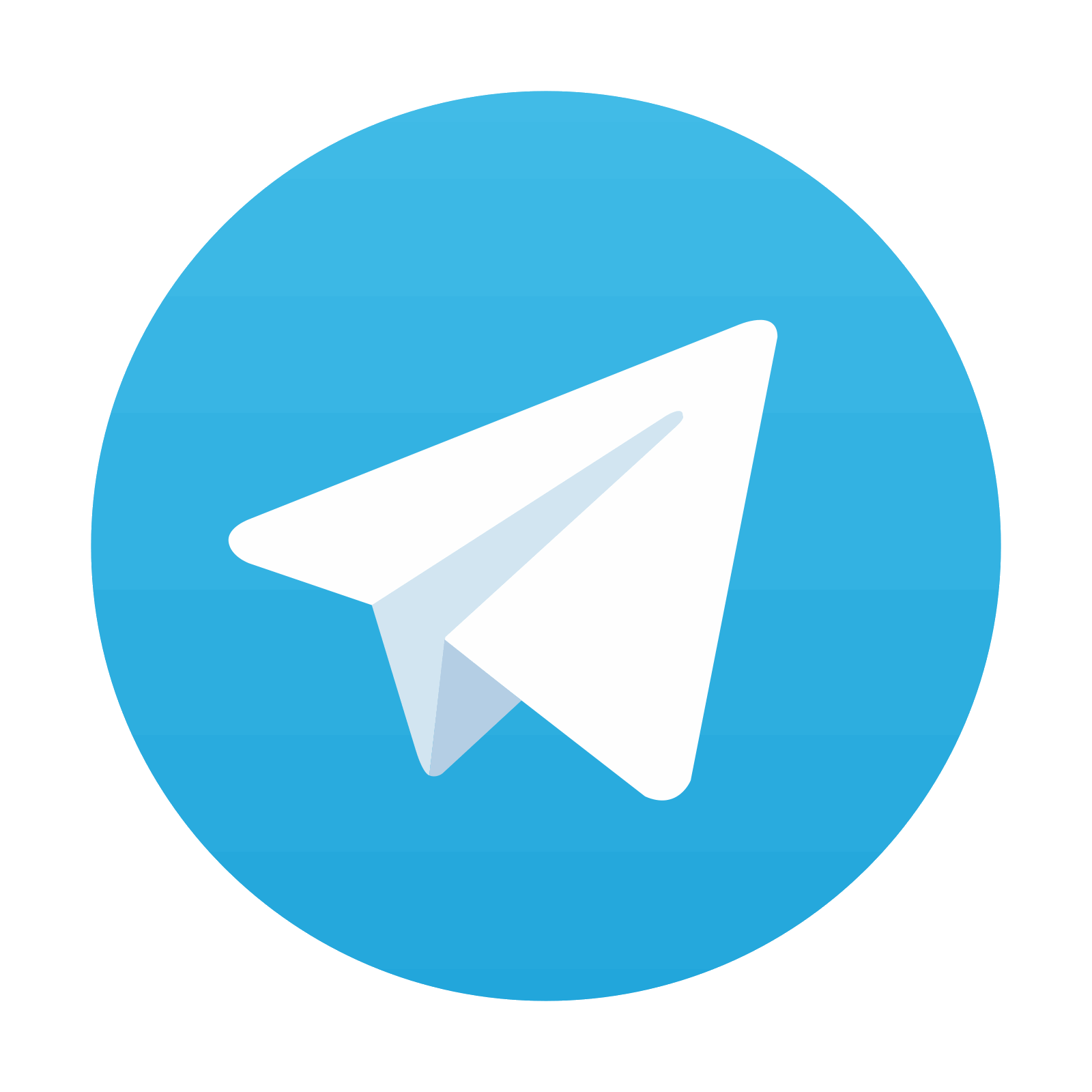
Stay updated, free articles. Join our Telegram channel
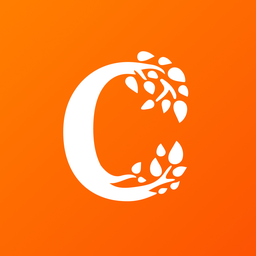
Full access? Get Clinical Tree
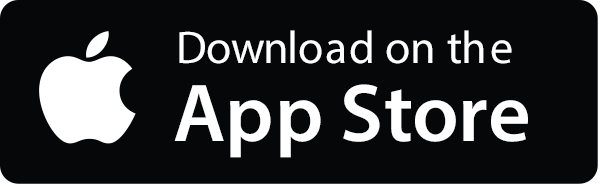
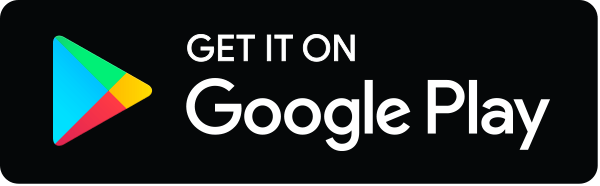