Category
Subtype
Wound characteristics
Mechanical
Abrasions
Contusions
Lacerations
Incisions
Puncture wounds
Removal of superficial skin layer(s)
Disruption of blood vessels and extravasation of blood into tissue
Tissue disruption caused by blunt or sharp instrument, usually irregular
Tissue disruption caused by sharp instrument, usually linear
Penetration of sharp instrument or projectile into tissue
Thermal
Superficial
Partial thickness
Full thickness
Burns confined to epidermis
Burns involving papillary (superficial) or reticular (deep) dermis
Burns extending through dermis into subcutaneous tissue
Chemical
Alkali
Acid
Hydrocarbons
Fat saponification, cellular dehydration, and deep tissue penetration
Hard eschar, thermal injury, and electrolyte imbalances
Dissolution of cell membranes, typically superficial erythema & blistering
Electrical
Complex
Degrees of cutaneous and deep tissue injury associated with systemic complications
Radiation
Complex
Basal skin layer damage with short- and long-term sequelae
Chronic
Complex
Persistent inflammation and matrix degradation leading to nonhealing
Mechanical injuries take on a variety of forms. Abrasions are caused by a friction event such as scraping or rubbing and result in the removal of superficial skin layers. More severe forms of abrasions include avulsions, which involve detachment of skin and possibly underlying tissue. Degloving injuries are avulsions with compromised blood supply to the detached. Contusions, or bruises, are caused by blunt trauma and characteristically rupture blood vessels. Extravasation of blood into the affected tissue is evident by skin discoloration, which evolves over time as the hemoglobin degrades. Lacerations and incisions refer to tissue separation extending through the skin, with lacerations caused by accidental trauma and incisions caused by purposeful dissection. Puncture wounds result from sharp penetration through the skin by an instrument or a projectile. They may extend into deeper structures and/or produce a second wound at the exit site (through-and-through wounds).
In the mid twentieth century, Jackson described thermal injuries as demonstrating a characteristic cutaneous injury pattern, which is divided into three zones based on blood perfusion and tissue viability: zone of coagulation, zone of stasis, and zone of hyperemia. The innermost zone of coagulation represents the irreversibly damaged, necrotic tissue without perfusion. Surrounding the necrotic tissue is an area of moderately burned tissue that may survive or progress to coagulative necrosis depending on the wound environment. This so-called zone of stasis is characterized by increased capillary permeability and vascular damage. The final zone of hyperemia is an area of intense vasodilatation and inflammation that contains viable tissue and is not usually at risk of progression to necrosis [7, 8].
Electrical injuries produce a variety of cutaneous and extracutaneous damage that depend upon the strength (amperage), duration of contact, and path of transmission through the body. If the contact time is brief, damage is relatively restricted to the cell membrane and electrical mechanisms (e.g., heart arrhythmias), rather thermal mechanisms will dominate the injury pattern. With longer contact time, thermal injury dominates and the entire cell is affected. Higher amperage (charge per unit time) burns are associated with a greater degree of systemic complications such as ventricular fibrillation, rhabdomyolysis , compartment syndrome, and renal failure [9].
Chemical injuries are subdivided by causative agent into alkali and acid, with alkali burns generally considered the most severe. Alkali burns induce fat saponification (calcification) or liquefaction, profound cellular dehydration, and formation of alkaline proteinates (completely ionized proteins) that cause deeper tissue damage. Examples of alkalis include lime, cement, potassium hydroxide, and bleach. Acid injuries induce protein hydrolysis (tanning) and do not penetrate tissue as readily as alkalis. One notable acid burn results from hydrofluoric acid due to its unique mechanism of fluoride chelating calcium in the tissue and the risk of hypocalcemia; this is the only acid burn with a specific treatment with topical or systemic calcium. Finally , hydrocarbons such as organic solvents are capable of dissolving cell membranes and producing skin necrosis. Systemic absorption of hydrocarbons is associated with respiratory depression and hepatic toxicity [10].
Radiation with energy high enough to break up molecules is called ionizing radiation. Radiation injuries, which can be accidental or iatrogenic, are known to cause short- and long-term sequelae. Acute radiation syndrome describes the adverse effects of large doses of ionizing radiation on the skin. Basal skin layer damage results in inflammation, erythema, and desquamation. Blistering and ulceration may follow in days to weeks, and most wounds will heal normally, though larger doses may result in destruction of skin appendages, fibrosis, abnormal pigmentation, and ulceration or necrosis of exposed tissue. Acute ionizing radiation exposure is also associated with dysfunction of hematopoietic, gastrointestinal, and cerebrovascular, and systems [11].
32.3 Mechanisms of Wound Healing
Wound healing is classically divided into four phases: hemostasis, inflammation, proliferation, and remodeling. Considerable overlap exists between each phase, and a combination of biochemical and cellular events contributes to the natural continuum of tissue repair.
32.3.1 Hemostasis
The initial phase of wound healing is characterized by a coordinated effort between circulating platelets, soluble clotting factors, and vascular endothelium to stop hemorrhage by the formation of a clot. The key sequences of events are divided into (1) coagulation cascade and (2) platelet activation, although it is important to remember the fundamentally integrated nature of these processes.
Hemostasis is initiated by a chain reaction of soluble serum proteins to form an insoluble fibrin mesh. The coagulation cascade is grouped into the contact activation and tissue factor pathways (historically the intrinsic and extrinsic pathways, respectively). The initial reactions of the two enzyme cascades are unique with a final common pathway consisting of factors X, V, and thrombin. The primary pathway for blood coagulation is the tissue factor pathway, with the contact activation pathway playing a secondary role. The clotting cascade results in the generation of fibrin, which enhances platelet aggregation, and structurally reinforces the ensuing platelet plug [12]. Topical fibrin sealants promote hemostasis and skin graft adhesion in excised burn wounds [13].
Disruption of normal endothelium exposes subendothelial collagen and thrombogenic extracellular matrix molecules, most notably von Willebrand factor (vWF). Platelets adhere to vWF via the glycoprotein (GP) Ib receptor, which strengthens the interaction between platelets and underlying extracellular matrix. Patients with vWF deficiency or with mutations in the glycoprotein (GP) Ib receptor are known to have von Willebrand disease, which is the most common hereditary coagulation deficiency. Likewise, mutations in the GPIb receptor result in Bernard–Soulier syndrome. Both of these conditions result in bleeding tendencies because of altered platelet adhesion to exposed subendothelium [14].
Platelet adhesion leads to platelet activation, invoking the release of stored granule contents. Cues from the wound environment such as hypoxia and acidosis enhance platelet degranulation [15]. Alpha-granules store growth factors such as platelet factor-4 (PF4), platelet derived-growth factor, fibronectin, vWF, and fibrinogen [16]. Many of these substances enhance platelet adhesion or activation. PF4 binds with high affinity to endothelial-derived heparin, which inactivates the molecule and promotes coagulation. Antibodies bind to the PF4-heparin complex on platelet membranes in the syndrome of heparin-induced thrombocytopenia (HIT), which can lead to dangerously low levels of platelets with a paradoxical increase in thrombosis [17].
Dense granules harbor smaller molecules involved in platelet activation such as ADP, ATP, calcium, and serotonin. Release of these molecules into the platelet cytosol initiates a Gq-linked protein receptor cascade, which results in an increased cytosolic calcium concentration.
The platelet glycoprotein IIb-IIIa receptor deserves mention because of its relevance to cardiovascular medicine and disease. The natural ligand of GPIIb-IIIa is fibrinogen, which links the coagulation cascade with platelet activation. Platelet activation leads to increasing its affinity to bind fibrinogen, which enhances platelet aggregation and clotting factor-mediated coagulation. The activated platelets change shape from spherical to stellate, and the fibrinogen cross-links with glycoprotein IIb-IIIa receptors in neighboring platelets to promote aggregation and eventual clot formation [18]. The GPIIb-IIIA receptor is the target of several antiplatelet agents including abciximab, eptifibatide, and tirofiban. Similarly, the drug clopidogrel is known to inhibit ADP binding to the GPIIb-IIIA receptor, which results in a reduced ability of platelets to aggregate and consequently form clots.
32.3.2 Inflammation
Vasoconstriction occurs at the wound site immediately after injury, which is the beginning of the second event in wound healing: inflammation. Vasoconstriction is primarily mediated by catecholamines (epinephrine and norepinephrine), prostaglandin F2α, and thromboxane A2. The contraction of blood vessels aids platelet aggregation and hemostasis. Vasoconstriction is followed shortly by vasodilatation and increased vascular permeability, which allows access of inflammatory cells to the damaged tissue. Vasodilatation is mediated by prostaglandin E2, prostacyclin, histamine, serotonin, and kinins [16]. Inflammatory cells undergo a three-stage process of rolling along the vascular endothelium, integrin-mediated adhesion to endothelial cells, and transmigration into the extracellular space [19].
Neutrophils are the first inflammatory cell to arrive at the wound and play a primary role in the phagocytosis of bacteria and tissue debris. A huge array of molecular signals are chemoattractant agents for neutrophils, including products of platelet degranulation, formyl methionyl peptides cleaved from bacterial proteins, and the degradation products of matrix proteins. Neutrophils are a major source of early cytokines in the systemic response to injury, including tumor necrosis factor (TNF)-α [20].
Oxygen delivery influences all stages of wound healing. In addition to providing a substrate for ATP synthesis in aerobic cell metabolism, large quantities of oxygen are used by neutrophils for superoxide radical generation in oxidative killing. Furthermore, molecular oxygen itself is toxic to anaerobic microorganisms, and reactive oxygen species are used as chemotactic and extracellular signaling including phyagocyte recruitment in the healing wound bed [21]. Wound oxygenation is determined by blood perfusion, hemoglobin dissociation, local oxygen consumption, fraction of inspired oxygen, hemoglobin content, arterial oxygen tension, circulating blood volume, cardiac output, arterial inflow, and venous drainage [22].
Another early cell to infiltrate the wound site are monocytes, which undergo phenotypic changes into macrophages. Macrophages can be regarded as a “master cell” involved in wound healing because of their central role in phagocytosis, inflammatory cell recruitment, and systemic inflammation. Macrophages release a variety of growth factors such as transforming growth factor-β (TGF-β), platelet-derived growth factor (PDGF), and fibroblast growth factor (FGF), which induce fibroblast proliferation and extracellular matrix production [23]. Macrophages express specific receptors for IgG (Fc receptor), complement C3b (CR1 and CR3), and fibronectin (integrins) that facilitate recognition and phagocytosis of opsonized pathogens. Importantly, macrophages also secrete cytokines such as IL-1 and TNF-α that modulate the systemic response to injury. Excessive production of TNF-α has been linked with multisystem organ failure as well as chronic non-healing ulcers [24]. As discussed later, both IL-1 and TNF-α appear to play crucial roles in early wound healing but may have an inhibitory effect on wound maturation if persistently elevated.
Emerging data suggest a role for nerve-derived neuropeptide in wound repair. Stimulation of efferent nerves is known to produce local vasodilation and plasma extravasation in skin, which contributes to the local inflammatory response. The neuropeptide substance P, released from terminal endings of sensory nerves in response to noxious stimuli, is known to influence inflammatory cell chemotaxis [25, 26], angiogenesis [27, 28], and keratinocyte proliferation [29]. We have previously suggested that the dysregulated neuroinflammation plays an important role in hypertrophic scarring, evident by increased levels of substance P and decreased levels of the regulating enzyme neutral endopeptidase [30], which is responsible for the exuberant matrix production, hyperemia, and pruritus seen in this condition [31].
A robust but appropriate inflammatory response is essential to prepare the wound bed for subsequent migration of proliferative cells. However, overzealous inflammation may inhibit the formation of granulation tissue and neovascularization. Experiments in mice constitutively expressing the chemotactic cytokine interferon-inducible protein 10 demonstrate that an intense inflammatory infiltrate inhibits angiogenesis and development of healthy granulation tissue [32]. Thus, as in all homeostatic systems, a careful balance of functionalized cellular and biochemical processes is essential to proper wound healing. Animal trials suggest a possible role for statins in wound healing in partial- and full-thickness burns. Whereas the combination of statins and angiotensin receptor antagonists have been demonstrated to reduce fibrosis in several organ systems, results in burn healing has been mixed. Atorvastatin has been shown to reduce inflammation and increase graft take in porcine wounds; however, in the same animal model, Losartan treatment resulted in decreased graft take, increased wound contraction and worse scarring [33, 34].
32.3.3 Proliferation
The proliferative phase is characterized by the formation of granulation tissue, which is a pink, soft, highly vascularized platform for tissue formation. Fibroblasts are evident at the wound site within 2–5 days and become the predominant cell type after the first week [35]. Macrophages drive the migration of fibroblasts by secreting a number of chemokines including TNF-α, PDGF, FGF, and TGF-β. Fibroblasts begin to deposit collagen and other extracellular matrix molecules that strengthen the wound bed. Macrophages stimulate fibroblasts to produce FGF-7 (keratinocyte growth factor) and IL-6, which promote keratinocyte migration and proliferation. IL-6 is also a potent stimulator of fibroblasts, which explains the decreased level found in aging fibroblasts and fetal wounds [36]. Unfortunately, granulation tissue also harbors high bacterial counts and proteolytic activity, so it may need to be excised before skin grafting. Granulation tissue in a burn wound prevents advancement of the epithelial tongue and epithelialization and likely leads to hypertrophic scar formation [37].
A number of other inflammatory cytokines may find clinical relevance in wound care. IL-8 secreted by macrophages and fibroblasts early in wound healing may have a stimulatory effect on keratinocytes and epithelialization. Topical application of IL-8 to human skin grafts in a chimeric mouse model enhanced keratinocyte proliferation and re-epithelialization [38]. Additionally, in both human and animal studies, topical application of PDGF improved wound strength and healing time [39]; unfortunately, clinical trials have demonstrated no significant difference in healing in chronic wounds [40].
TGF-β is expressed by platelets and fibroblasts in the wound bed and plays an important role in collagen deposition and turnover. TGF-β is the most potent known stimulator of fibroblast proliferation and can accelerate wound healing in steroid-treated and irradiated animals [41]. Overexpression of TGF-β mRNA has been found in keloid and hypertrophic scars, whereas fetal wounds contain relatively little TGF-β [42]. This contrast between the heavily fibrotic scars of keloid and the scarless repair observed in utero might underscore the importance of TGF-β in the fibrotic response to tissue injury. Gabriel [43] observed a similar phenomenon in burn injuries, where higher levels of TGF-β correlate with excessive wound contraction. Interestingly, exogenous application of TGF-β3 reduces monocyte and macrophage recruitment to the wound site, resulting in less deposition of collagen and fibronectin in the early stages of wound healing [44]. A formulation of TGF-β (Juvista) underwent phase III trials but failed to meet primary or secondary endpoints and was never widely released [45].
Coincident with fibroblast migration to the wound site is angiogenesis. Angiogenesis, or neovascularization, which has been thought to be a critical element of early wound healing for transport of metabolites to and from the regenerating tissue. More recent data suggest that normal healing can occur when angiogenesis is inhibited and that angiogenesis in the wound bed is not associated with increased blood flow to the wound. Vascular endothelial cells arise from both preexisting blood vessels and endothelial progenitor cells (EPCs) in bone marrow. The most important regulators of angiogenesis are vascular endothelial growth factor (VEGF) and FGF-2. Nissen et al. [46] observed a dose-dependent effect of both VEGF and FGF-2 in angiogenesis. VEGF is secreted as many different isoforms from a variety of stromal and mesenchymal cells, with the tyrosine kinase VEGF-receptor 2 emerging as the most preeminent in angiogenesis. VEGF/VEGFR2 signaling is involved in EPC migration from bone marrow, as well as promotion of endothelial cell proliferation and differentiation [47].
Hypoxia is a potent inducer of both angiogenesis and fibroblast proliferation. The major player in hypoxic gene expression is hypoxia-inducible factor 1 (HIF-1), a DNA-binding transcription factor that is known to alter gene transcription of a number of proteins involved in metabolism, angiogenesis, migration, and proliferation [48]. Cultured endothelial cells upregulate the expression of several pro-angiogenic molecules when cultured in hypoxia, including endothelin-1, VEGF, and PDGF-β chain [49]. Fibroblast replication and longevity are increased in low oxygen tension culture [50], as is TGF-β secretion [49]. These observations highlight the contribution of hypoxia in the wound bed in proliferative cell signaling.
Mechanotransduction or the translation of mechanical force into biochemical signaling represents one novel area of research [51]. Using matrix-integrin activation of signaling cascades, mechanical force is transmitted to targets including calcium-dependent signaling, nitric oxide signaling, mitogen-associated kinases, and Rho GTPases. The end product of these signals activate transcription factors that move to the nucleus and activate mechanoresponsive genes [51]. Silicone sheeting, a way of offloading skin tension in healing wounds, has shown promise in improving scaring. Further avenues to modulate the biochemical signaling and mechanotransduction networks have potential to reduce scar formation and promote skin regeneration [52, 53].
Recently, the role of immune cells, long ignored in wound healing research is under increased investigation. T cells migrate into the wound bed during the late proliferative and early remodeling phase. Mice deficient in T and B cells have a reduced capacity to scar [22], though contradictory reports exist concerning the beneficial effects of CD4+ and CD8+ lymphocytes on wound healing [54, 55]. Additionally, a unique type of T cell exists in the skin, known as dendritic epidermal T cells (DETC), which are thought to modulate many aspects of wound healing such as inflammation, host defense, and maintenance of tissue integrity. Mice lacking or defective in DETC show a delay in wound closure and a decrease in keratinocyte proliferation at the wound site [44, 56].
32.3.4 Epithelialization
Epithelialization is the third important response to cutaneous injury, critical because once the epithelial layer is regenerated the wound is often viewed as being “healed.” Keratinocytes migrate from wound edges and dermal appendages such as hair follicles, sweat glands, and sebaceous glands. The role of the epidermal appendages is especially evident in partial-thickness burns. Since the advancing epithelial tongue at the wound edge can migrate no more than ~1 cm, wounds depend on epidermal sources at the center of the wound. Full-thickness wounds larger than 2 cm rarely heal other than by contraction. Subsequent proliferation of these cells at the wound site provides a neo-epidermal covering. Keratinocyte migration and proliferation follow a discrete sequence of events: disassembly of hemidesmosomes and desmosomes , retraction of intracellular tonofilaments and keratin filaments, and formation of focal contacts and cytoplasmic actin filaments [57]. Martin [58] has extensively studied the interplay between laminin, matrix metalloproteinases (MMPs), integrins, and soluble growth factors in this process.
Renewal of keratinocytes during normal homeostasis and wound repair is a defining feature of re-epithelialization. The upper region of hair follicles below the sebaceous gland (known as the bulge) contains multipotent progenitor cells that contribute to maintenance and renewal of epithelium [59, 60]. Additionally, epidermal cells migrate from neighboring unwounded epidermis or from the infundibulum, the portion of the hair follicle between the epidermis and the sebaceous gland [61].
The relative contributions of the follicular stem cells and epidermal stem cells to re-epithelialization is debated, although genetic analyses have confirmed that the epidermis has intrinsic capacity for self-renewal and does not depend on follicle-derived multipotent progenitor cells [62, 63]. Further evidence for this notion comes from reports of de novo hair follicle generation in healing skin of adult mice [64]. This phenomenon, which has never been observed in humans, is contingent upon WNT-mediated signaling which is also involved in pattern formation and the epithelial–mesenchymal transformation during embryogenesis [65]. Elucidation of the overlapping pathways in wound repair, and development is a central principle of efforts toward scarless repair and skin regeneration.
32.3.5 Remodeling
Remodeling is the replacement of granulation tissue with scar over months after “healing.” A key feature of tissue remodeling that emerges during this stage of wound healing is the balance between extracellular matrix (ECM) synthesis and degradation. While fibrogenic growth factors such as PDGF and FGF stimulate fibroblast matrix deposition, resident cells induce continuous degradation by matrix metalloproteinases. MMPs are a family of zinc proteases that are capable of degrading a variety of ECM components such as collagen, fibronectin, proteoglycans, and laminin [66] which can improve wound healing through direct and indirect mechanisms [67].
Collagen composition of the wound appears to follow a similar pattern as embryogenesis. Granulation tissue is comprised of a large amount of collagen III, which is gradually replaced by collagen I. Collagen I provides a higher degree of tensile strength to the developing scar, although the final tensile strength approaches only 70% of uninjured skin [68]. A morphological change in fibroblasts ensues during wound contraction, in which fibroblasts begin to express alpha-smooth muscle actin and adapt functions of smooth muscle cells. The resulting cell is termed a myofibroblast and serves to enhance wound contraction.
32.3.6 Stem Cells
In addition to resident epidermal stem cells in the skin, bone marrow-derived stem cells may contribute substantially to cutaneous wound healing. Bone marrow contains both hematopoietic (CD34+) and non-hematopoietic (mesenchymal) stem cells which aid wound healing by direct contribution of cells as well as by paracrine signaling. A notable study, in which green fluorescent protein-labeled bone marrow stem cells were used to reconstitute the marrow of mice with cutaneous wounds, indicated that non-hematopoietic mesenchymal stem cells may contribute up to 15–20% of dermal fibroblasts in normal skin and healing cutaneous wounds. Fathke et al. [69] and Brittan et al. [70] have traced cells with a keratinocyte phenotype to bone marrow origin. Deng et al. [71] have shown evidence that bone marrow stem cells are involved in hair follicle regeneration. Bone marrow cells expand ex vivo to promote neovascularization [72], appendage regeneration [73], and accelerate wound closure [74].
Endothelial progenitor cells (EPCs) derive from CD34+ hematopoietic stem cells in the bone marrow and contribute some proportion of the endothelial cells to adult skin. Systemic transplantation of EPCs enhances wound healing in mice [75], as does topical application of EPCs to ischemic ulcers in diabetic mice [76]. The mechanism involves paracrine signaling from EPCs instead of direct contributions by endothelial cells [75].
Fibrocytes, a subpopulation of leukocytes that also arise in the bone marrow, were originally identified by their rapid recruitment from peripheral blood to wound sites in mice [77]. Fibrocytes are significantly increased in the blood of burned patients in comparison to normal individuals and appear to localize in the deeper papillary dermis [78]. These cells may contribute to the myofibroblast population in wounds and may be associated with hypertrophic scarring [79, 80].
32.4 Abnormal Wound Healing
32.4.1 Impaired Wound Healing
Local and systemic factors that impair wound healing
Local factors | Systemic factors |
---|---|
Tension Foreign bodies Infection Ischemia Hematoma and seroma Trauma Edema Irradiation | Connective tissue disorders Hypothermia Oxygen Tobacco smoking Malnutrition Jaundice Age Diabetes mellitus Uremia Steroids Chemotherapeutic agents |
A variety of insults can disturb wound healing by evoking hypoxia in the evolving wound. Disruption of vascular supply and depletion of oxygen can lead to wound hypoxia, which is associated with systemic diseases such as connective tissue disorders and microvascular disease in diabetes mellitus. Tobacco smoking produces similar effects through nicotine-induced vasoconstriction and displacement of oxygen on hemoglobin with carbon monoxide [83].
Infection is another classic adversary of proper wound healing. Wounds with bacterial counts that exceeding 105 organisms per gram of tissue will generally not heal by any means, including flap closure, skin graft placement, or primary intention [84]. The introduction of early excision and grafting for burn wounds has significantly reduced the prevalence of burn wound sepsis—which was historically a leading cause of burn mortality. Endotoxin produced by gram-negative bacteria stimulates phagocytosis and collagenase expression, which contributes to matrix degradation and destruction of normal tissue. Bacteria also accelerate protease production in macrophages (such as MMPs) while inhibiting protease inhibitor expression. This effect leads to increased matrix destruction and degradation of growth factors, which are characteristics of chronic nonhealing wounds [85].
Nutritional status has a profound effect on wound healing as well. Serum albumin is one of the most accurate predictors of surgical morbidity and mortality, with levels below 2.1 g/dL associated with poorer outcomes [86]. Protein replacement enhances wound healing [87], as does supplementation with the amino acids arginine, taurine, and glutamine [88, 89]. Whereas patients with large burns characteristically have albumin levels below 1.0 g/dL, exogenous albumen has never been demonstrated to improve outcomes. Early introduction of nutrition is a critical component of acute burn care and wound healing, with micronutrients playing an important role [90, 91]. Data suggest that the catabolic state can be modulated by propranolol in children [92] and oxandrolone in children and adults [93, 94]. Propranolol, a nonselective β-blocker, improved wound healing and perioperative hemodynamics in severely burned adults by increasing vascular resistance in the burn beds by leaving α-adrenergic receptors unopposed and decreasing blood loss during operative interventions [95].
Vitamin C (ascorbic acid) is an essential cofactor in proline and lysine hydroxylation during collagen synthesis, and supplementation of 100–1000 g per day may improve wound healing [89]. Vitamin A (retinoic acid) is required for wound re-epithelialization, maintenance of normal epithelium, proteoglycan synthesis, and normal immune function. Oral retinoid therapy counteracts the detrimental effects of corticosteroids on wound healing, possibly through promotion of TGF-β and IL-1 signaling [96]. Vitamin K deficiency impedes clot formation and hemostasis, while vitamin D is required for bone healing and calcium metabolism. Finally, vitamin E supplementation may serve an important role as an antioxidant in trauma patients. Early administration of vitamin E reduces the incidence of organ failure and the average length of ICU stay in critically ill surgical patients and may be relevant for burn patients [97].
The dietary minerals associated with wound healing include zinc and iron. Zinc is an essential cofactor in RNA and DNA polymerases. Deficiency inhibits granulation tissue formation [98] and delays wound healing [99]. Desneves et al. [88] report that zinc supplementation improves wound healing. Iron, another cofactor in DNA synthesis, is also key to proline and lysine hydroxylation. Although the role of iron in normal hematopoiesis is well established, chronically anemic patients do not appear to suffer from delayed wound healing [100]. Whereas selenium deficiency causes hair and skin abnormalities in humans and selenoproteins have been implicated in keratinocyte function and cutaneous morphogenesis [101], but selenium deficiency is yet to be associated with abnormal wound healing.
32.4.2 Hypertrophic Scars and Keloids
Hypertrophic scar and keloids represent fibroproliferative scars, which are characterized by excessive collagen deposition. These two morphological aberrations are difficult to differentiate: keloids are defined as scars that grow beyond the periphery of the original wounds and hypertrophic scars represent raised scars that remain confined to the boundaries of the original wound. Keloids rarely regress with time; hypertrophic scars frequently regress spontaneously. Both scar types appear to have a strong genetic component, with more prevalence in dark-skinned patients of African, Asian, Native American, or Latin American descent. Hypertrophic scars, the result of prolonged inflammation, are influenced by wound depth, skin tension, and delayed wound healing with increased inflammatory response and enhanced fibroblast activity [102]. Though theoretically preventable, hypertrophic scars tend to occur in pigmented individuals [103], young people, and rarely in the aged. Interestingly, they often develop in highly contractible body sites and rarely form on the scalp or the lower leg [104]. Proposed therapies to control hypertrophic scars act to reduce inflammation including steroid injections, radiotherapies, compression, and surgical methods to reduce skin tension [105]. The single nucleotide polymorphism p27kip1 decreases vascular restenosis due to fibrogenesis, but was not associated with hypertrophic scar severity [106]. Using a genome-wide association study of over 500 individuals with burn injuries, mutations in the CSMD1 gene have been associated with reduced hypertrophic scarring [107].
In light of its potent effect on fibroblast proliferation and collagen deposition, it is perhaps not surprising that TGF-β plays a central role in proliferative scarring. Colwell et al. [108] found increased levels of the TGF-β1 isoform in both keloids and hypertrophic scars. Likewise, antibodies to TGF-β isoforms reduce fibrosis in hypertrophic scars [109]. Novel therapies for hypertrophic and keloid scars are in development that target ECM synthesis and fibroblast proliferation [110].
32.4.3 Chronic Nonhealing Wounds
Dysfunction of normal wound healing processes leads to chronic wounds. In particular, chronic wounds appear to have sustained inflammation with less matrix production. Chronic wounds exhibit higher levels of cytokines such as IL-1, IL-6, and TNF-α, with reduced levels of essential growth factors such as EGF and PDGF. MMP-1, MMP-2, MMP-8, and MMP-9 are present at higher levels, with reduced levels of MMP inhibitors [111]. These nonhealing wounds are prone to developing squamous cell carcinoma, originally reported in burn wounds by Marjolin. Marjolin ulcers tend to be very aggressive and should be highly suspected with nonhealing burn wounds [112]. Therapy includes complete local extirpation of the cancer with negative margins and lymphatic mapping [113]. Other conditions such as osteomyelitis, pressure sores, venous stasis ulcers, and hidradenitis have also been associated with wound malignancies [114]; patients with impaired skin integrity due to burn injuries are at increased risk for decubitus ulcers, which constitute a closely monitored hospital acquired complication.
32.4.3.1 Wound Dressings
Examples of burn dressings
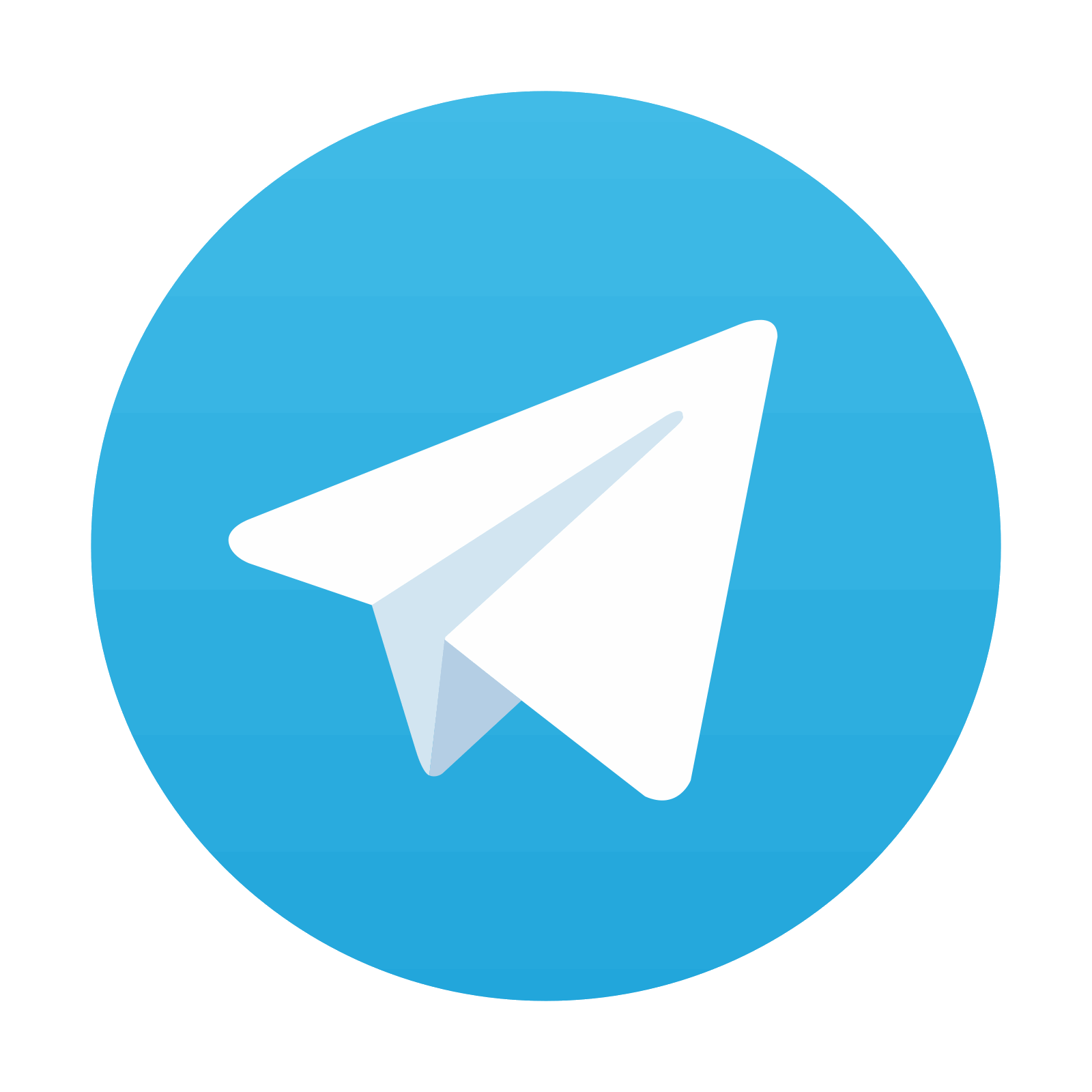
Stay updated, free articles. Join our Telegram channel
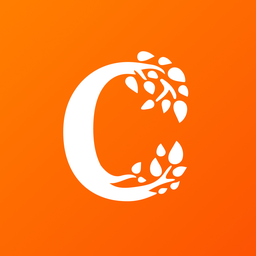
Full access? Get Clinical Tree
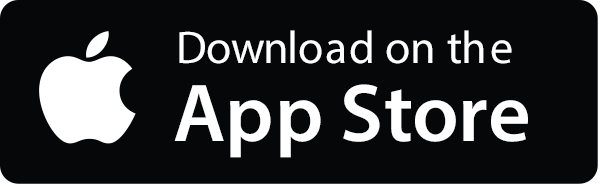
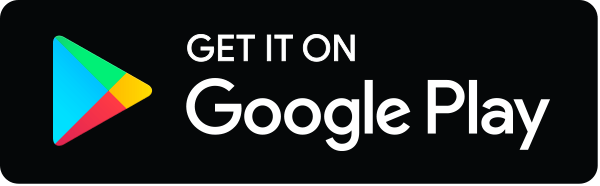