Under–resuscitation
Over–resuscitation
Oliguria < 0.3 mL/kg/h
Polyuria > 1.0 mL/kg/h
Hemoglobin > 18 g/dL (Hct > 55%)
Decreasing PaO2/FiO2, →pulmonary edema
Sodium > 145 mEq/L
Increasing pulmonary artery wedge pressure, central venous pressure (CVP)
Cardiac index < 2 L/min/m2
Rapidly increasing cutaneous edema
Mixed venous oxygen saturation (SvO2) < 55%
Fluid delivery > Ivy index (fluid delivery > 250 mL/Kg body weight) [30]
Plasma lactate > 2 mmol/L or increasing
Intra-abdominal hypertension
Base deficit < −5 mmol/L or decreasing
Compartment syndrome
Adequate fluid resuscitation (e.g., Parkland 4 cc/kg/m2 burn of Ringer’s lactate)
Albumin substitution after 8–12 h after burn if initial fluid resuscitation fails (5% albumin 75–125 cc/h)
Transfusion of packed red blood cells
Dobutamine if low cardiac index (0.5–1 μg/kg/min)
Norepinephrine or epinephrine in cases of fluid-resistant hypotension
Vasopressin in refractory distributive shock (up to 0.03 units/min)
Nitroprusside (up to 10 μg/kg/min)
Labetalol (10–20 mg)
Nicardipine (5 mg/h)
In patients with associated trauma and altered mental status that exhibit unexplained early arterial hypertension, the presence of traumatic brain injury should be considered.
Rates of infusion during resuscitation tend to be attenuated following hour 13 after injury. Even when comparing different resuscitation strategies, Salinas et al. showed a sustained increase in urine output after the 12 h mark [28]. This effect, might be secondary to a relative and progressive myocardial recovery. After 24–48 h, the patients generally become spontaneously hyperdynamic and the fluid delivery should be drastically reduced to about 30–40% of that infused during the first 24 h. As the microvasculature starts to seal and peripheral blood flow is increased to the burned areas, the physiology shifts to a decreased vascular permeability, increased heart rate, and decreased peripheral vascular resistance resulting in an increase in cardiac output. The patients’ metabolic rate is increased to nearly three times that of their basal metabolic rate [31].
20.2.1.1 Crystalloids
Adequate resuscitation is a key element of early burn critical care [15–19]. Maintenance of organ perfusion during burn shock depends upon the restoration of intravascular volume. The principles of burn fluid resuscitation were developed in the early 1950s. The most common algorithm, the Parkland formula, calculates a total volume of crystalloid to be given over the first 24 h according to 4 mL × weight (kg) × TBSA (%) [15, 17, 27, 32, 33]. Drs. Baxter and Shires published their seminal article in 1968 after experiments on animal models followed by their early experience with burn patients. They used animal models to determine direct extracellular fluid volume changes in the early post-burn period. Subsequently, 11 patients were treated experimentally with the volumes estimated by the experimental burns. They proposed that in the first 24 h post-burn, hemodynamics appear to be closely in correlation with functional extracellular fluid volume maintenance but unrelated to volume thereafter. Even in this early work, significant changes in cardiac output were noticed in burn subjects [34].
In accordance with the American Burn Association, the resuscitation formula is only to be used as a guideline for resuscitation in burn shock [16–18, 26, 33]. The endpoints (urine output of 0.5 cc/kg/h, MAP > 60) which traditionally had been used for fluid resuscitation are not always adequate. With the advent of goal-directed therapy (base deficit, lactate, cardiac index and/or output, and stroke volume variation) [15, 27, 32, 33, 35, 36], it has become apparent that traditional formulas may inadequately estimate fluid requirements. The Parkland formula is deficient in calculating the fluid requirements for resuscitation in patients with: a large burn size, deeper burns, an inhalation injury, delays in resuscitation, current alcohol or drug use, and an electrical injury, leading to inadequate/inappropriate resuscitation.
Patients with severe burns and electrical injuries receive far greater crystalloid volumes than predicted, resulting in “fluid creep” [16, 17, 27, 36, 37] with its inherent complications such as: pulmonary edema, pleural effusions, pericardial effusions, abdominal compartment syndrome, extremity compartment syndrome, and the conversion of burns to deeper wounds. In addition, increasing the fluid administration in burned patients significantly increases the risk of developing acute respiratory distress syndrome (ARDS), pneumonia, bloodstream infections, multiorgan failure, and death [26]. Given the risk of abdominal compartment syndrome with large burns and its dire consequences, intra-abdominal pressure monitoring is therefore recommended in burns involving more than 30% TBSA [30].
The selection of crystalloid for resuscitation has been debated extensively. Exudates and edema fluid are known to be isotonic, containing the same amounts of electrolytes and protein as plasma. Whatever the formula, the fluid used must contain sodium in sufficient concentration to deliver about 0.5 mmol/kg/%TBSA by 48 h to prevent water intoxication associated with hypotonic fluids. Ringer’s lactate solution is a common option. However, the use of other alternatives is acceptable. The use of normal saline can induce acidemia due to the disassociation of ions of sodium and chloride in the solution, further exacerbating the acidosis associated with burn injury. Hypertonic saline has been associated with hypernatremia, renal failure, and mortality when used in burn resuscitation.
By day 3, the interstitial fluids that have accumulated during the first 24–48 h start to mobilize and be excreted. This might be augmented by active stimulation of diuresis using loop diuretics, sometimes in combination with an aldosterone antagonist.
As the resuscitation phase approaches an end, it becomes important to determine the total daily maintenance fluid requirement, which can be calculated by:
![$$ \mathrm{Basal}\kern0.5em \mathrm{Fluid}\ \left(1500\kern0.5em \mathrm{mL}\times \mathrm{BSA}\left[{\mathrm{m}}^2\right]\right)+\mathrm{Evaporative}\kern0.5em \mathrm{Water}\kern0.5em \mathrm{Loss}\ \left(\right[\left(25+\mathrm{TBSA}\left(\%\right)\Big]\times \mathrm{BSA}\left[{\mathrm{m}}^2\right]\times 24\kern0.5em \mathrm{h}\right) $$](/wp-content/uploads/2020/11/188491_2_En_20_Chapter_TeX_Equa.png)
However, calculated fluid balances are difficult to calculate, as they do not account the exact amount of exudative losses through the burn wounds (about 0.5–1.0 L/10% TBSA/day). The condition may be complicated by the use of fluidized or air beds, which cause an even greater loss of free water.
20.2.1.2 Colloids
The use of albumin in burn patients is not well defined and to date no prospective randomized trial in burn patients shows an advantage or disadvantage of albumin administration for burn resuscitation, maintenance, or burn infection/sepsis [17, 38]. The use of albumin and its timing is controversial. Many burn care providers believe that albumin has a positive effect in the case of burn resuscitation as a rescue modality. The use of colloids reduces the amounts of total fluids administered, decreasing the risks of over-resuscitation and consequently decreasing the risk of intra-abdominal compartment syndrome. The use of albumin has been related to decreased mortality and a decreased incidence of extremity compartment syndrome and renal failure [39–41]. Huzar et al. has proposed the use of thrombelastography (TEG) to predict patients who will require supranormal resuscitation. The risks of over-resuscitation have been described elsewhere in this chapter but the early identification of these patients might prompt an earlier use of colloids [42].
To the contrary, Cochrane systematic reviews show no benefit in mortality with colloids over crystalloids alone. No difference in outcomes was seen among the many colloid solutions; moreover, the use of hydroxyethyl starch might increase mortality [43, 44]. The use of TEG and thromboelastometry (ROTEM) can aid in the decision of which colloid to use, and fresh frozen plasma (FFP) could be favored in patients with a concurrent hypocoagulable state [42]. The use of FFP is associated with transfusion-associated lung injury and anaphylaxis. Since colloids are substantially more expensive than crystalloids and might have potentially fatal complications, their use should be individualized. In patients with comorbidities sensitive to fluid changes, such as renal or cardiac insufficiency, the use of colloids is justified. Some have proposed the protocolized use of albumin in patients with hypoalbuminemia (<2.0 g/dL) or in those for whom resuscitation is failing [45].
20.2.1.3 Transfusion
Transfusion guidelines are currently under investigation. Transfusion requirements should be aligned with a patient’s operative plan and rehabilitation efforts. If significant burn excision is planned, the patient ought to be optimized and a low starting hemoglobin concentration is not recommended. During the rehabilitation phase, anemia can compromise activity levels. The results of delayed or impaired rehabilitation are not inconsequential in this patient group [46].
A target level of at least 7 g/dL is reasonable, but if a patient is premorbid with impaired cardiac function or poor oxygen delivery, we consider reaching hemoglobin levels of 8 mg/dL. A recent prospective randomized multicenter trial contrasted a liberal transfusion strategy with a restrictive modality and found that with a restrictive approach, the blood product utilization halved. Major outcomes evaluated, such as bloodstream infection, mortality, and organ dysfunction, were not worse than the liberal strategy [47].
20.2.1.4 Vasopressors/Inotropes
Vasopressors or inotropes can be used when indicated in difficult to resuscitate patients. Usually during the first 8–12 h, vasopressors should be avoided as vasoconstriction can have adverse effects. The evidence in favor of using one vasopressor or a combination over others is scarce in the literature and continues to be debated. Titration is usually dependent on mean arterial pressure with a goal of greater than 60 mmHg, though even this can be debated. Normal filling pressures should not be an objective, as this strategy causes over-resuscitation and its multitude of subsequent complications. A study by Evans et al., based on an animal model, showed that the potency of α-adrenergic receptor agonists to increase mean arterial pressure within 24 h after burn is reduced, and the potency and efficacy of a vasopressin receptor agonists are increased. Severe burn sensitizes intrinsic vasopressin receptor reactivity in resistance arteries. While this data might assist in selecting a vasopressor drug, it is unknown if early use of vasopressors will positively impact outcomes [48].
Dobutamine as an inotrope can improve cardiac function if cardiac output or cardiac index is low (<3). The classic vasopressors, epinephrine and norepinephrine, should be used with caution. Vasopressin is currently being studied in various trials, and we await the results. In the critical care population, vasopressin did not improve outcomes compared to catecholamines. In addition, there are case reports that show no benefit with vasopressin but an increased incidence of adverse effects, which is usually associated with high doses of vasopressin (>2.4 IU). However, it appears that doses between 1.2 IU and 2.4 IU are relatively safe and can improve blood pressure to meet goals. The use of vasopressin as a second-line agent has also been suggested. Dopamine, another inotropic agent, is used by some but generally is not widely used in burns.
20.2.2 Invasive and Noninvasive Monitoring
Monitoring of vital signs and other measures in large burns is crucial. Resuscitation endpoints, including clinical, hemodynamic, and biochemical parameters, might improve outcomes of resuscitation. The exclusive use of formulas can be misleading as fluid requirements can be impacted by other factors such as burn depth, inhalation injury, associated injuries, age, a delay in resuscitation, escharotomies/fasciotomies, and the use of alcohol or drugs [16].
Another study evaluating base deficit and lactate levels during burn resuscitation confirmed increased mortality with increased base deficit and lactate derangement. The study authors noted that the normalization of base deficit within 24 h after burn correlated with improved outcomes compared to patients with prolonged elevated base deficit [49]. These findings have been corroborated in other studies in burned patients [50, 51]. Therefore, increased tissue hypoxia may represent under-resuscitation and result in increased organ dysfunction and higher mortality.
Pulse contour analysis is based on the shape of the arterial waveform from either a femoral or radial arterial catheter. Prospective data regarding pulse contour analysis in burn patients are currently lacking. Transesophageal echocardiography, partial carbon dioxide rebreathing, and impedance electrocardiography are some of the other methods used to determine cardiac output. Tissue perfusion monitors, such as gastric tonometers and subcutaneous devices, have been tested without substantial clinical application.
Cardiovascular monitoring requirements will depend on the extent of injury and the presence of inhalation injury. Volume status and cardiac performance are especially difficult to evaluate in the burned victim. In particular, burned extremities may impede the ability to obtain a blood pressure reading by a sphygmomanometer (blood pressure cuff). Generally, in cases with burns over 20% TBSA an arterial catheter will be used for blood pressure monitoring, in those patients who are intubated due to inhalation injury or airway protection. Blood gas determinations will, in addition, enable the monitoring of arterial lactate and the evolution of acid-base status. With increasing severity of burns and in patients over 60 years, information about cardiac output becomes more valuable.
Hemodynamic findings in early invasive cardiovascular monitoring will be variable. Massive burns are generally associated with cardiogenic shock even in the youngest patients and will invariably be present in the elderly during the early phase. The etiology includes increased afterload caused by high levels of stress hormones, direct depression of the myocardium by cytokines, lipoperoxides, intravascular hypovolemia with low preload, and vasoplegia [25, 52].
Pulmonary catheters may be used in patients with massive burns and major cardiopulmonary comorbidities. Invasive hemodynamic monitoring via a pulmonary artery catheter allows the direct and continuous measurement of central venous pressure (CVP), pulmonary capillary wedge pressure, cardiac output, systemic vascular resistance, oxygen delivery, and oxygen consumption. Pulmonary artery catheter-guided therapy has been studied most extensively in trauma and critically ill surgical patients. Hemodynamic data derived from the pulmonary artery catheters appears to be beneficial to ascertain cardiovascular performance in certain situations (inadequate noninvasive monitoring, difficulty in defining endpoints of resuscitation). The cardiac output and cardiac index are useful in determining cardiac sufficiency. Pulmonary capillary wedge pressure and systemic vascular resistance are markers of volume status and shock. Potential complications of pulmonary artery catheter placement include arrhythmias, blood clots, and blood vessel damage. However, the general practicability, risk-benefit ratio, and lack of mortality reduction when using a pulmonary artery catheter have been widely criticized. At the moment, there are no studies in burned patients to provide evidence-based recommendations. In order to overcome the disadvantages of the pulmonary artery catheters, less invasive techniques have been developed.
A novel approach for burn patients has been the use of thermodilution catheters to determine cardiac function, resistance, and lung water [18, 53, 54]. The use of these catheters may enable focused and algorithm-driven therapy that may improve the resuscitation phase, but currently, only a few small studies have been published, which does not allow major conclusions to be drawn; these catheters however do show promise for optimizing resuscitation [18].
Transpulmonary thermodilution can provide similar information to a pulmonary artery catheter, with the use of a central line and an arterial line. With transpulmonary thermodilution (TPTD), a cold saline bolus is injected into the central venous circulation, and the subsequent change in blood temperature is measured by a thermistor-tipped arterial catheter. This is connected to a commercially available device (PiCCO) that calculates flows and volumes from the dilution curves. In addition to cardiac output and systemic vascular resistance measurement, TPTD allows an estimation of global end-diastolic volume and intrathoracic blood volume, both of which are indicators of cardiac preload, and extravascular lung water, a marker of pulmonary edema. The use of TPTD goal-directed therapy based on intrathoracic blood volume and extravascular lung water measurements in critically ill patients has been studied in various prospective trials and shows promising results. The evidence for the use of TPTD is contradictory but the obtained data are reproducible and have a reasonable correlation with data obtained from pulmonary artery catheters [54].
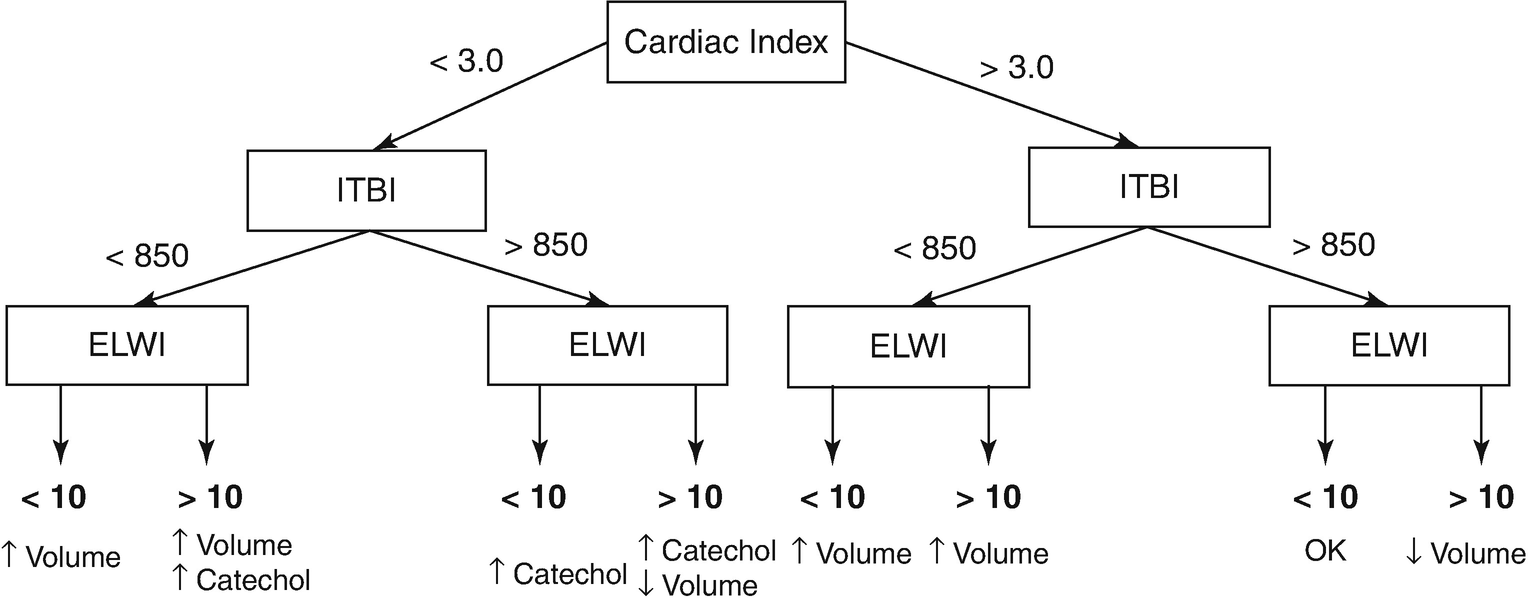
Algorithm for fluid resuscitation based on transpulmonary thermodilution parameters. ITBI intrathoracic blood volume index, ELWI extravascular lung water index
Other methods, such as pulse contour analysis and transesophageal echocardiography (TEE), are alternatives for determining hemodynamic parameters [55], while subcutaneous gas tension and gastric tonometry have been used as markers of resuscitation.
20.2.2.1 Urine Output
Hourly urine output (UOP) continues to be the most commonly used endpoint for resuscitation. We have limited evidence that other methods, more invasive or more expensive, can result in better outcomes [56].
Urinary output in the acute phase of a burn is indicative of adequate organ perfusion and its suggested target is 0.5–1.0 mL/kg/h. In children, urine output is targeted to 1 mL/kg/h. Certain situations, including electrical or crush injury with associated rhabdomyolysis, merit additional monitoring and fluid loading [46]. However, urine output is not always an adequate indicator and can be affected by the burn itself, infusion of antioxidants during resuscitation, cardiac or renal comorbidities, and central or peripheral renal insufficiency.
20.2.2.2 Central Venous Pressure
CVP is a rough marker for preload and hence filling of the patient. CVP should be measured correctly at the level of the heart with a subclavian or jugular line in place. The range of an adequate CVP in burned adults is 4–8 mmHg, and 2–6 mmHg in burned children. The use of CVP as an endpoint for resuscitation has been challenged as it can be highly influenced by external or abdominal pressures, limiting its reliability to monitor intravascular volume [57].
20.2.3 Intra-abdominal Hypertension and Abdominal Compartment Syndrome
With overenthusiastic fluid delivery, increasing intra-abdominal pressures (IAP) and consequently abdominal compartment syndrome (ACS) becomes a matter of concern in both adult and pediatric burn patients [30, 58]. The Ivy index (250 mL/kg fluid resuscitation) is a cut-off value beyond which trouble is nearly certain [30].
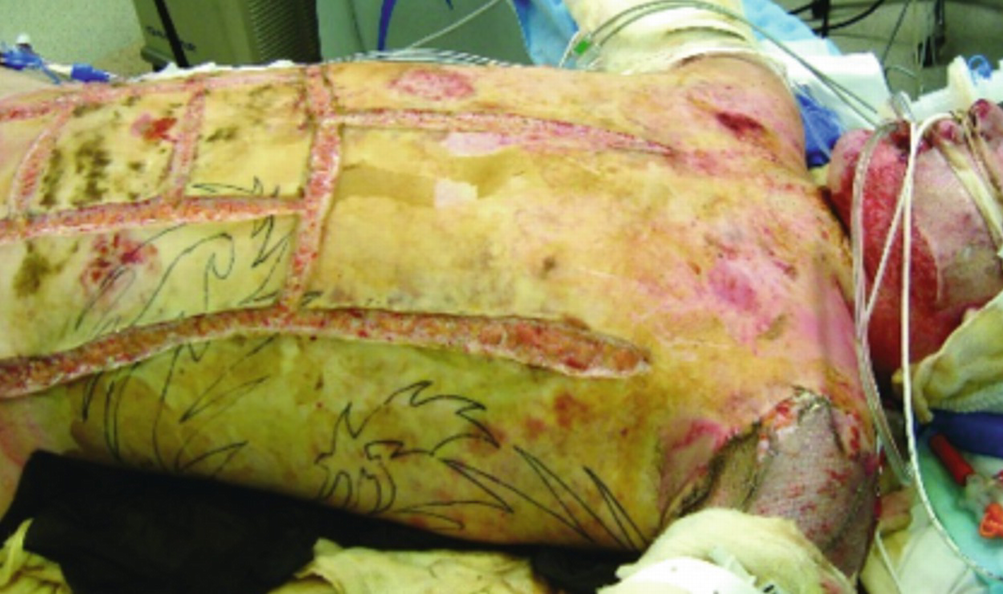
20.2.4 Respiration
Indications for intubation
PaO2 < 60 mmHg |
PaCO2 > 50 mmHg (acutely) |
P/F ratio < 200 |
Impending respiratory/ventilatory failure |
Severe upper airway edema |
Severe facial burn |
Burns over 40% TBSA |
Clinical signs of severe inhalation injury: edema, blisters, or ulceration during laryngoscopy |
Effective gas exchange should be determined in an arterial blood gas analysis. Oxygen saturation should be over 85%. The respiratory rate should be 8–20 in adults and 14–38 in children.
20.2.4.1 Ventilation Settings
Detailed descriptions of the different ventilation modes are beyond the scope of this text. In short, positive end-expiratory pressure (PEEP) is useful in supporting oxygenation. The level of PEEP required should be established by empirical trials and reevaluated on a regular basis. PEEP levels should start at 5 cm H2O and be increased in 2–3 cm increments. PEEP trials should be done to optimize oxygenation and cardiac output. The effectiveness of continuous positive airway pressure or PEEP is related to the surface tension abnormalities and the marked tendency for atelectasis in these patients.
Pressure control ventilation with permissive hypercapnia is the current preferred method of treatment for ventilated patients. In general, plateau pressures above 35 cm H2O are concerning but in conditions where there is a decreased chest wall compliance as in patients with extensive burns, greater ranges might be acceptable. To accomplish the goal of limiting plateau pressures, pCO2 can be permitted to rise (permissive hypercapnia) unless other contraindications exist that demand a more normal pCO2 or pH.
If pulmonary edema continues, the amount of PEEP and of oxygen should be elevated to maintain adequate gas exchange. Low-tidal volumes (5–8 mL/kg) with PEEP may be needed to improve oxygenation. In general, peak flow rates should be adjusted as needed to satisfy patient inspiratory demands. As for the inspiratory/expiratory (I:E) ratio, the inspiratory time should be long enough to deliver the tidal volume at flow rates that will not result in airway turbulence and high peak airway pressures. The normal I:E ratio is 1:2. This may be adjusted to increase the ratio if oxygenation becomes difficult. With inspired oxygen concentration as a starting point and until the level of hypoxemia is determined, a patient placed on a ventilator should receive an oxygen concentration of 100%. Decrease the FiO2 as ABG improves. Keep in mind that chest and abdominal wall burns can result in poor compliance and high inspiratory pressures.
In efforts to choose a ventilation mode that is lung protective but at the same time appropriately oxygenates and ventilates, nonconventional modes are often used. A low-tidal volume strategy in burn patients with inhalation injury can be challenging as there is a restrictive and obstructive pathophysiology. Chest wall eschar, edema, and bulky dressings as well as bronchospasm and debris-related bronchial obstruction contribute to this effect.
High-frequency percussive ventilation (HFPV) is a time-cycled, pressure-limited mode of ventilation that delivers sub-tidal volumes at high rates. Tidal volumes are determined by peak inspiratory pressure settings and volume provided by oscillatory function. The use of HFPV is typically restricted to a rescue modality for oxygenation failure although some have reported that use of this strategy as a primary mode for those with inhalation injury results in decreased use of rescue modes. It has been used in adult ARDS but its application in the burn/inhalation injury population is limited. HFPV has demonstrated improved oxygenation at lower peak airway pressures when compared to a low-stretch strategy. The percussive effect in HFPV is thought to facilitate the evacuation of airway debris and mucous plugs. In a randomized controlled trial, Chung et al. concluded that no significant difference between HFPV and low-tidal volume modes with respect to the lung protection provided with a decreased use of rescue modalities [63].
The experience with extracorporeal membrane oxygenation (ECMO) for burn patients is scarce but it can be useful in selected cases that have failed other modes with refractory hypoxemia [64].
Extubation criteria
PaO2/FiO2 ratio > 250 |
Maximum inspiratory pressure > 60 cm H2O |
Spontaneous tidal volume > 5–7 mL/kg |
Spontaneous vital capacity > 15–20 mL/kg |
Maximum voluntary ventilation > twice the minute volume |
Cough peak flow > 60 L/min |
None to moderate amount of secretions (suction frequency) |
Audible leak around the endotracheal tube with deflated cuff |
Adequate secretions management (cough/gag reflexes) |
20.2.4.2 Tracheostomy
Tracheostomy is safe in burn patients. The decision to perform one should be weighed with the timing of the grafting of the neck. There is no consensus on timing. Patients with major burns and significant inhalation injury will likely benefit the most as they will undergo multiple surgical interventions and lengthy mechanical ventilation. In patients with damage to the vocal cords secondary to the inhalation injury, tracheostomy should be considered to prevent further damage to the vocal cords and airway.
20.2.5 Inhalation Injury
Inhalation injury is a significant confounder in burn injury, increasing morbidity and mortality. Of all major burns, approximately 20–30% are associated with a concomitant inhalation injury, with a mortality of 25–50% when patients undergo ventilatory support for more than 1 week after injury [3, 5, 66]. However, the incidence of inhalation injury is typically co-linear with burn size and severity, decreasing its use as a predictor of outcomes.
Inhalation injury can be classified into three types: thermal injury (mostly limited to the upper airway), chemical injury of the respiratory tract and systemic toxicity due to metabolic asphyxiants, or a combination of these insults [67]. The severity of inhalation injury is directly proportional to early oxygenation challenges, complex hospitalization, increased fluid resuscitation requirements, ventilation demands, and mortality [68].
A significant portion of fire-related deaths result not from cutaneous burns, but from inhalation of the toxic products of combustion [33, 66, 69, 70]. Many of these compounds may act together, increasing mortality. This is especially true of carbon monoxide (CO) and hydrogen cyanide, where a synergism has been found that increases tissue hypoxia and acidosis in addition to decreasing cerebral oxygen consumption and metabolism. Carbon monoxide levels higher than 15–20% should be treated with 100% endotracheal oxygen. The use of hyperbaric oxygen is controversial and its availability is restricted [71].
Cyanide can be the result of the burning of many household products, especially those that contain cotton, wool, and nylon. Cyanide toxicity associated with inhalation injury remains a diagnostic dilemma, as markers for cyanide toxicity (elevated blood lactate, elevated BD, or metabolic acidosis) can also represent under-resuscitation, associated trauma, CO poisoning, or hypoxia. Regardless, aggressive resuscitation and administration of 100% oxygen remains a mainstay of treatment. Controversy remains as to the need for specific antidotes in cyanide poisoning [72]. The use of hydroxocobalamin (a standard in prehospital care in some European centers) has not been as widely accepted in North America. A retrospective review conducted in a center in the United States determined that its use was associated with a lower rate of pneumonia, faster effective extubation, and a decrease in intensive care unit stay when compared with controls [73]. There is minimal evidence for the role of cyanide antidotes in smoke inhalation injury; therefore, aggressive supportive therapy aimed at allowing for the hepatic clearance of cyanide without specific antidotes should be the first line of treatment.
Other possible contributing toxic substances are hydrogen chloride (produced by polyvinyl chloride degradation), nitrogen oxide, and aldehydes, which can result in pulmonary edema, chemical pneumonitis, or respiratory irritability. Direct thermal damage to the lung is seldom seen except as a result of high-pressure steam, which has 4000 times the heat-carrying capacity of dry air. Laryngeal reflexes and the efficiency of heat dissipation in the upper airway prevent heat damage to the lung parenchyma.
First stage: Acute pulmonary insufficiency. Patients with severe lung injuries show acute pulmonary insufficiency from 0 to 36 h after injury with asphyxia, carbon monoxide poisoning, bronchospasm, upper airway obstruction, and parenchymal damage.
Second stage: Pulmonary edema. This second stage occurs in 5–30% of patients, usually from 6 to 72 h post burn and is associated with a high mortality rate.
Third stage: Bronchopneumonia. This appears in 15–60% of these patients and has a reported mortality of 50–86%. Bronchopneumonia occurs typically 3–10 days after burn injury and is often associated with the expectoration of large mucus casts formed in the tracheobronchial tree. Early pneumonias are usually due to penicillin-resistant Staphylococcus species, whereas after 3–4 days, the change of flora on the burn wound is reflected in the appearance in the lung of Gram-negative species, especially Pseudomonas species.
A history of exposure to smoke in a closed space
Altered mental status (stuporous or unconscious patients)
Facial burns, singed nasal vibrissae, bronchorrhea, sooty sputum, wheezing or rales on auscultation
Laboratory findings of hypoxemia, elevated levels of carbon monoxide
Chest X-ray findings. Can be an insensitive method because they may remain normal as long as 7 days post burn.
Bronchoscopy. Should be the standard diagnostic method on every burn patient.
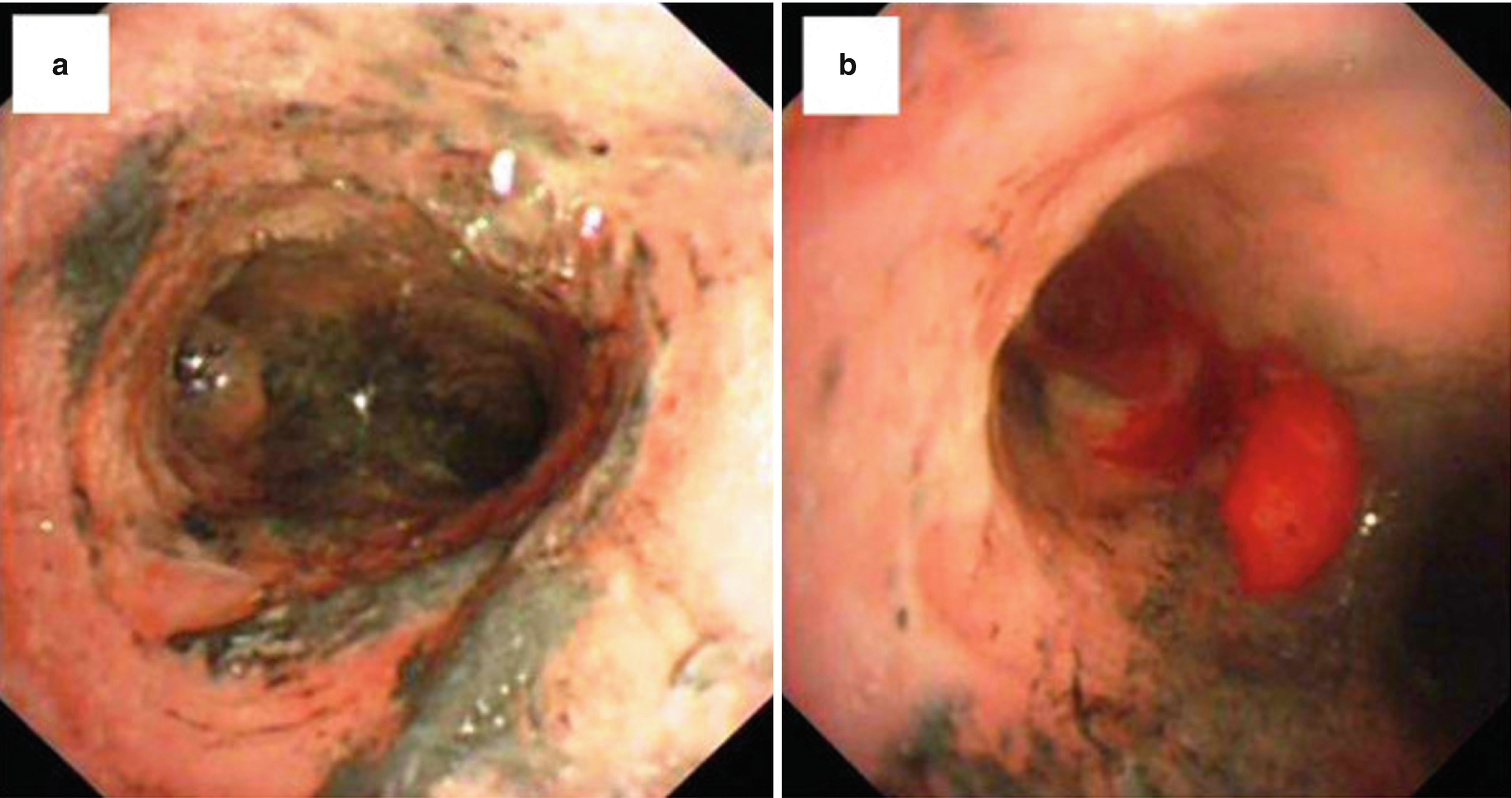
(a) Trachea with severe edema, erythema, and carbon soot deposition. (b) Formation of pseudomembrane [75]
Inhalation injury scale
Grade 0 (no injury) |
Absence of carbonaceous deposits, erythema, edema, bronchorrhea, and obstruction |
Grade I (mild injury) |
Minor or patchy areas of erythema, carbonaceous deposits in proximal or distal bronchi (any one of these or a combination) |
Grade II (moderate injury) |
Moderate degree of erythema, carbonaceous deposits, bronchorrhea with or without compromise of the bronchi (any one of these or a combination) |
Grade III (severe injury) |
Severe inflammation with friability, copious carbonaceous deposits, bronchorrhea, bronchial obstruction (any one of these or a combination) |
Grade IV (massive injury) |
Evidence of mucosal sloughing, necrosis, endoluminal obliteration (any one of these or a combination) |
The treatment of the inhalation injury should be started immediately, with the administration of 100% oxygen via a face mask or nasal cannula. This serves to reverse the effects of carbon monoxide poisoning and aids in its clearance, as 100% oxygen lowers its half-life time from 250 to less than 50 min. The role of hyperbaric oxygen remains controversial although both physiological data and some randomized trial data suggest a potential benefit in terms of a reduction in cognitive sequelae; hence, it has been included in the guidelines of the Undersea and Hyperbaric Medical Society [71, 76].
Several clinical studies have shown that pulmonary edema was not prevented by fluid restriction, as the interstitial fluid after thermal and inhalation injury is caused mainly by the inflammatory process [77]. Although overhydration could increase pulmonary edema, inadequate hydration increases the severity of pulmonary injury by the sequestration of polymorphonuclear cells and leads to increased mortality.
Prophylactic antibiotics for inhalation injury are not used, but are clearly indicated for documented lung infections. Empiric choices for treatment of pneumonias prior to culture results should include coverage of methicillin-resistant Staphylococcus aureus in the first few days after burn (these infections develop within the first week after burn) and of Gram-negative organisms (especially Pseudomonas or Klebsiella), which mostly occur after 1 week after burn. Systemic antibiotic regimes are based on serially monitored sputum cultures, bronchial washings, or transtracheal aspirates.
Inhalation injury results in airway obstructive casts, which are composed of mucus secretions, denuded airway epithelial cells, inflammatory cells, and fibrin [11]. The presence of fibrin solidifies the airway content forming the firm cast that is hard to remove by a single cough or even by aggressive airway toilet. Therefore, prevention and dissolution of airway fibrin deposition is crucial in effective airway management.
Inhalation injury is one of the most important predictors of morbidity and mortality in burn patients. When present, inhalation injury increases mortality up to 15 times [33, 66, 72, 78]. Inhalation injury prompts endotracheal intubation, which in turn increases the incidence of pneumonia. Pneumonia can increase mortality in up to 60% in these patients. Patients usually recover full pulmonary function and late complications are not the rule. In patients who have been recently extubated, cough after swallowing might represent an early sign of a tracheo-esophageal fistula. Scarring of the airway can cause stenosis, rupture, and dysphonia, requiring voice therapy and occasionally surgery.
20.2.5.1 Pharmacological Management
Bronchodilators (albuterol/salbutamol) for smooth muscle relaxation and bronchospasm inhibition
Muscarinic antagonists (tiotropium) to decrease airway pressures and mucus secretions
Nebulized heparin to inhibit cast formation controlling fibrin creation
A systematic review in 2014 highlighted proof of the potential benefits of inhaled heparin and similar alternatives. Inhaled anticoagulation regimens in both preclinical and clinical studies improve survival and decrease morbidity without altering systemic markers of clotting and anticoagulation. In some preclinical and clinical studies, inhaled anticoagulants were associated with a favorable effect on survival [79]. A multicenter randomized controlled trial by Glas et al. [80] is currently underway to assess nebulized heparin versus placebo in inhalation injury, and it is expected to be completed by the end of 2017.
Nebulized acetylcysteine, a mucolytic with anti-inflammatory properties that attenuates reactive oxygen species damage
Nitric oxide to improve oxygenation
Nitric oxide has been used to improve oxygenation in treating hypoxic pulmonary vasoconstriction and to improve ventilation/perfusion mismatches and therefore tissue oxygenation. However, Enkhbaatar and colleagues have reported increased levels of nitric oxide in lung tissue secondary to inhalation injury and that the resultant loss of hypoxic vasoconstriction worsens ventilation-perfusion mismatch [81].
Hypertonic saline to induce effective coughing
Nebulized racemic epinephrine, which has been shown to be safe to use with no significant resultant hemodynamic changes. It reduces mucosal edema and produces bronchodilation [82].
Inhaled epoprosprostenol, which is used mainly in ARDS and decreases pulmonary artery pressure, produces vasodilation, and inhibits platelet aggregation. It results in decreased ventilation-perfusion mismatch [83].
Corticosteroids are contraindicated. The theoretical benefits of corticosteroid therapy include a reduction in mucosal edema, reduced bronchospasm, and the maintenance of surfactant function. However, in several animal and clinical studies, mortality increased with the administration of corticosteroids, and bronchopneumonia showed more extensive abscess formation.
20.2.6 Immune Response and Inflammation
The inflammatory response constitutes an organized defense mechanism aimed at protecting the body from further damage, restoring homeostasis, and promoting wound repair. This includes a local reaction to injury, a systemic response, massive cytokine production, immune and endocrine changes, increased protein catabolism, and the rearrangement of hepatic synthesis priorities. Cytokine production is strongly enhanced after major burns, and the balance between pro-inflammatory and anti-inflammatory mediators in acute injury is lost [84]; the phenomenon further increases infectious complications. The intensity of this reaction is correlated with mortality [85, 86]. Although inflammation is perceived as beneficial, its persistence for long periods of time can cause various derangements in the normal physiology. These metabolic and inflammatory changes can persist for up to 3 years. The changes can result in insulin resistance, increased fracture risk, growth and development retardation, increased cardiac work and cardiac dysfunction development, impaired strength and muscle function, hormonal abnormalities, and an increased risk for infections and sepsis. All the aforementioned can lead to organ dysfunction and failure, possibly resulting in mortality for the patient [87].
In healthy subjects, the endogenous antioxidant defense mechanisms are sufficient to cope with moderate free radical overproduction. In major burns, these defenses are overwhelmed, leaving space for the deleterious effects of reactive oxygen species, with proximity oxidation of nucleotides, proteins, and lipids. The endogenous antioxidant defenses become acutely depleted by the massive production of reactive oxygen species derived from both oxygen and nitric oxide.
The pro-inflammatory cytokines (particularly IL-6) are responsible for the redistribution of micronutrients (including those with antioxidant properties) from the circulating compartment to tissues and organs with high synthetic and cell replication activity.
20.2.7 Serum Organ Markers
Cardiac markers: troponin, A- and B-natriuretic peptide, CK
Liver: AST, ALT, bili, ALKP
Pancreas: amylase, lipase
Kidney: BUN, creatinine
Hematology: CBC including coagulation, differential including bands
Hormonal: cortisol including ACTH challenge, thyroid axis, GnRH
For longitudinal observation, it is recommended to obtain admission values and measures values once or twice per week.
20.3 Late Hospital Phase
The later phase includes performing critical practices to maintain organ function, control infection and sepsis, and alleviate hypermetabolism. This section will focus on maintaining organ function and on the complications of long-term ICU sequelae, as infection and sepsis and hypermetabolism are discussed in detail in later chapters.
20.3.1 Central Nervous System
Anoxic brain injury was a leading cause of death in burn patients, which has been replaced by sepsis and multiple organ failure [14]. Adequate resuscitation and early intubation have improved mortality in burn patients [19, 33]. However, neurological disturbances are commonly observed in such patients. The possibility of cerebral edema and raised intracranial pressure must be considered during the early fluid resuscitation phase, especially in the case of associated brain injury or high voltage electrical injury. The inhalation of neurotoxic chemicals, of carbon monoxide, or hypoxic encephalopathy may adversely affect the central nervous system [33, 66, 69, 72]. Other factors include hypo-/hypernatremia, hypovolemic shock, sepsis, antibiotic overdosage (e.g., penicillin), and possible oversedation or withdrawal effects from sedative drugs. If increased intracranial pressure is suspected, neurosurgery consultation needs to be considered and intracranial pressure monitoring and therapy initiated.
In general, severe burn injury is associated with nonspecific atrophy of the brain that normally resolves over time, and no intervention is needed. Hung et al. suggested that burned patients have a higher risk of ischemic stroke that persists for more than 1 year after injury [88].
20.3.1.1 Pain, Sedation, and Delirium
Pain and anxiety will generally require rather large doses of opioids and sedatives (benzodiazepines mainly). Continuous infusion regimens will generally be successful in maintaining pain within acceptable ranges. Sedation and analgesia should be assessed frequently. In the case of analgesia, for patients with intact communication skills, self-reporting scales are preferred. If the patient has a limited ability to use a self-reporting numeric rating scale (NRS), scales like the Behavioral Pain Scale (BPS) and Critical Care Pain Assessment Tool (CPOT) are available. Sedatives can be targeted using appropriate scores on the Richmond Agitation Sedation Scale (RASS) or Riker Sedation Agitation Scale scores (SAS). Thus, the sequelae associated with oversedation and opioid creep are prevented, namely fluid creep and effects on the central and peripheral cardiovascular system [37]. Consideration should be given to the use of NMDA receptor antagonists, such as ketamine or gabapentin, which have important opioid-sparing effects in order to decrease the need for opioids and benzodiazepines [3, 5]. Multimodal pain management combining a long-acting opioid for background pain, a short-acting opioid for procedures, an anxiolytic, an NSAID, acetaminophen, and gabapentin for neuropathic pain control [3, 5] targeted to the Riker Sedation Agitation Scale and SAS scores and Behavioral Pain Scale scores should provide adequate analgesia and sedation.
Delirium is commonplace in mechanically ventilated patients and patients with prolonged hospital stays. Its presence results in longer hospitalization and increased mortality and costs. Patients can experience long-term cognitive impairment. Patients should be monitored for delirium frequently using the Confusion Assessment Method for ICU (CAM-ICU) or the Intensive Care Delirium Screening Checklist. Methods to prevent and treat delirium include early mobility and rehabilitation, sleep hygiene, adequate oxygenation, and the limited use of benzodiazepines.
20.3.1.2 Intensive Care Unit-Acquired Weakness
Survival and organ function have been the main outcome measures for burn patients; however, recently long-term outcomes have been the focus of burn care providers. Long-term outcomes include those of the peripheral nervous system and muscular system, derangements of which can manifest as neuromyopathy. In the burn population, the incidence of neuromyopathy is approximately 2–29%. Frequently, the first sign can be difficulty weaning the patient off mechanical ventilation [89]. The importance of positioning and the prevention of peripheral nerve compression is well known and ingrained in the daily practices of most critical care units. The main risk factors for neuropathy include multiple organ failure, muscular inactivity, prolonged periods of ventilation, hyperglycemia, and the use of corticosteroids and neuromuscular blockers. In a recent publication by de Jonghe et al. [90], early identification and treatment of conditions leading to multiple organ failure (especially sepsis and septic shock), avoiding unnecessary deep sedation and excessive hyperglycemia, promoting early mobilization, and weighing the risk and benefits of corticosteroids might reduce the incidence and severity of ICU-acquired weakness.
20.3.1.3 Thermal Regulation
Temperature regulation is altered with a “resetting” of the hypothalamic temperature above normal values [91–93]. The teleological advantage of maintaining an elevated core temperature following burn injury is not fully understood, but major burns destroy the insulating properties of the skin, while the patients strive for a temperature of 38.0–38.5 °C. Moreover, grafted skin has an impaired sweat response and cutaneous vasodilation, which persists to up to 8 years [94]. Sometimes, it is difficult to differentiate between elevated temperatures due to a central reset or due to other causes such as infection or fever. Cultures become useful if temperatures are persistently over 39 °C.
Catecholamine production contributes to changes associated with several cytokines, including interleukin-1 and interleukin-6. Any attempt to lower the basal temperature by external means will result in augmented heat loss, thus increasing the metabolic rate. The ambient temperature should be maintained between 28 and 33 °C to limit heat loss and the subsequent hypermetabolic response [4]. The metabolic rate is increased as a consequence of several factors such as the catecholamine burst, the thermal effects of pro-inflammatory cytokines, and evaporative losses from the wounds, which consumes energy, causing further heat loss. Evaporation causes extensive fluid loss from wounds, approximating 3750 mL/m2/%TBSA burns [3, 5]. Every liter of evaporated fluid corresponds to a caloric expenditure of about 600 kcal.
Besides hyperthermia, another very important contributor to poor outcomes is hypothermia. Burn patients frequently experience hypothermia (defined as a core temperature below 35 °C) on admission, in the ICU, during operations, and during sepsis [3, 5]. Time to recover from hypothermia has been shown to be predictive of outcomes in adults, with time to revert to normothermia being longer in non-survivors. Considering that hypothermia favors infections and delays wound healing, the maintenance of perioperative normothermia is of utmost importance. Tools for doing so include warming the ambient room temperature, intravenous fluid warming systems, and warming blankets. The temperature of the bed should be set at 38 ± 0.5 °C. However, this is contraindicated in the febrile patient, as it complicates fluid therapy due to largely unpredictable free water losses, and respiratory management due to the supine position. The patient may benefit from an additional 1–4 L of free water per day (as D5W IV or enteral free water) to prevent dehydration. These additional requirements are difficult to assess in the absence of bed-integrated weight scales.
20.3.2 Heart
Critically ill patients, including burn patients, are at a higher risk of developing in-hospital cardiac events, explained by the increased cardiac demand due to the injury burden and a hypermetabolic state. The adrenergic surge can trigger cardiac disturbances [95]. The burn population is unique as a consequence of the in-volume and electrolyte shifts. Arrhythmias are among the most common complications [96]. The resultant cardiomyopathy can require the use of inotrope therapy for maintaining end-organ perfusion.
Another complication that can occur is cardiac ischemia. Ischemic events can lead to a manifest heart or to temporary cardiac ischemia. If a myocardial infarction occurs, Cardiology consultation is especially beneficial if procedural intervention is required.
20.3.3 Lung
Pulmonary complications in the early phase are pulmonary edema and inhalation injury that were discussed previously. Pulmonary complications that occur during an ICU or hospital stay include ventilation-associated pneumonia (VAP)/pneumonia and acute respiratory distress syndrome (ARDS).
20.3.3.1 Ventilator-Associated Pneumonia (VAP)
Mechanically ventilated burn patients have a high incidence of VAP , which results in significant morbidity and mortality. VAP prevention should be a paramount feature of critical care of these patients. Preventative measures are usually grouped in bundles that include recumbent position and elevating the head, oral care, ulcer and deep venous thrombosis prophylaxis, subglottic suctioning, hand hygiene, daily spontaneous breathing trials, and daily sedation interruption [97]. The implementation of such bundles has produced a decreased risk of developing VAP in burn patients [98]. VAP early in admission is mostly caused by S. aureus, whereas those that occur late in the hospital course are caused by opportunistic bacteria such as methicillin-resistant S. aureus, P. aeruginosa, and A. baumannii [99].
Mechanically ventilated burn patients are at a high risk for developing VAP, with the presence of inhalation injury as a unique risk factor in this patient group.
VAP prevention strategies should be used in mechanically ventilated burn patients.
A clinical diagnosis of VAP can be challenging in mechanically ventilated burn patients where systemic inflammation and acute lung injury are prevalent. Therefore, a quantitative strategy, when available, is the preferable method to confirm the diagnosis of VAP.
An 8-day course of targeted antibiotic therapy is generally sufficient to treat VAP; however, resistant S. aureus and Gram-negative bacilli may require a longer treatment duration.
An effort should be made to reduce the ventilator days.
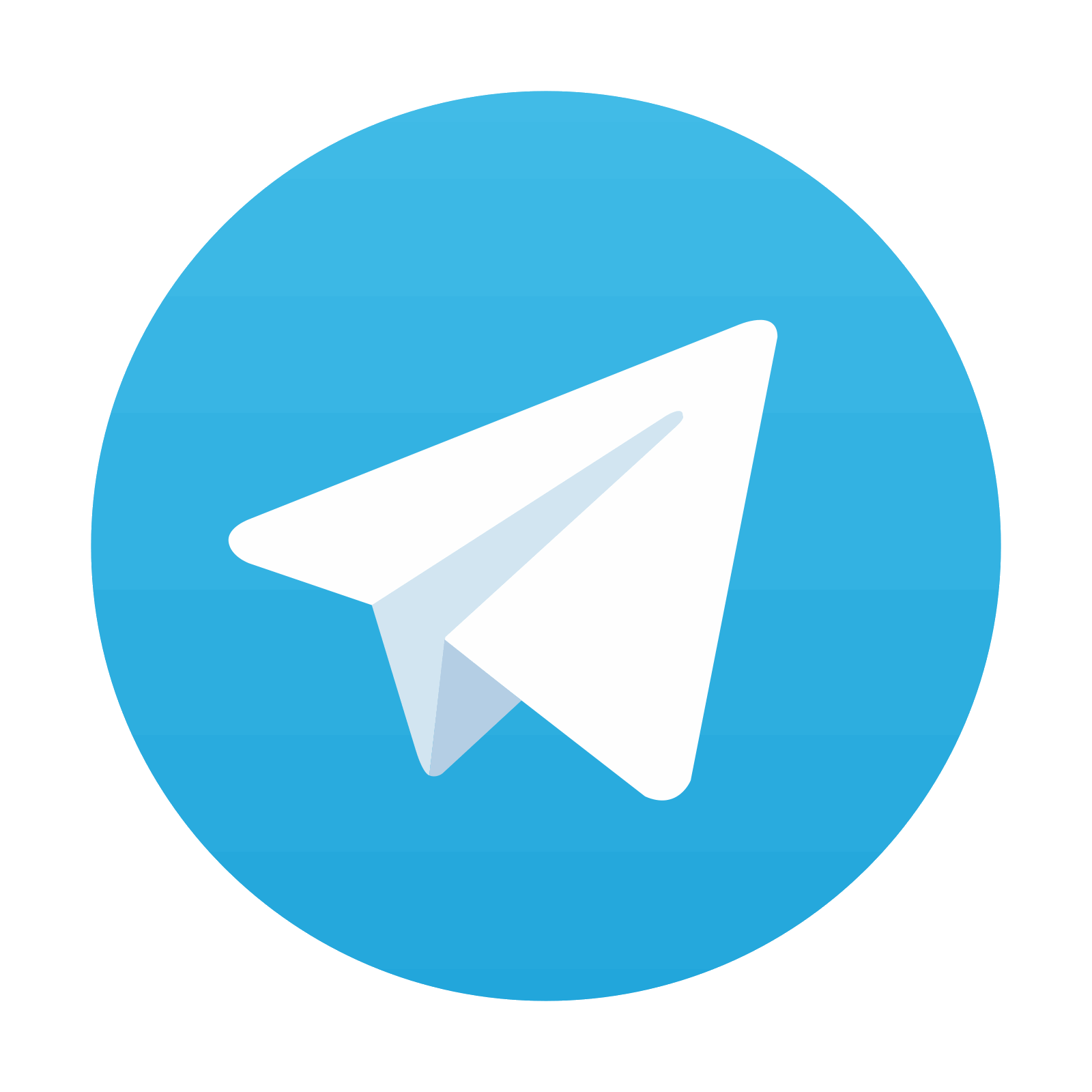
Stay updated, free articles. Join our Telegram channel
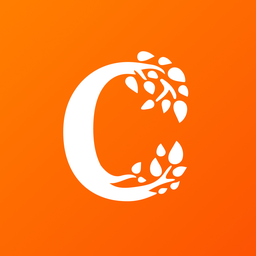
Full access? Get Clinical Tree
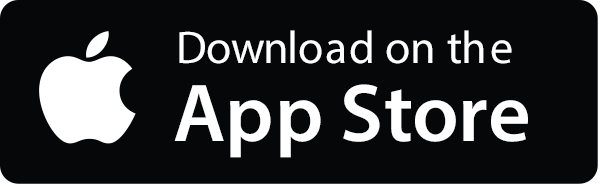
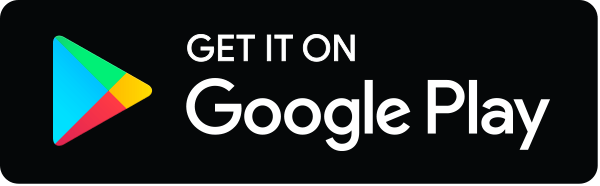