Protocol
Description
References
In vivo T cell depletion
6 Gy TBI
[48]
In vivo T cell depletion
Irradiation of the thymic/mediastinal area, reduction in TBI to 3 Gy
[99]
In vivo T cell depletion
Thymic irradiation combined with T-cell-depleting antibodies
[116]
In vivo T cell depletion
High dose of nonmyeloablative TBI—6.5 Gy
[5]
Co-stimulation blockers
Anti-CD40 ligand with or without CTLA4-Ig and 3 Gy TBI
Co-stimulation blockers
Anti-CD4 and anti-CD9 with anti-CD40 ligand
[26]
Administration of T-reg cells
T-reg cells administrated after myeloablative conditioning (8.5 Gy)
[40]
Administration of T-reg cells
T-reg cells combined with BMT, depleting antibodies and TBI (10 Gy)
[83]
In vivo T cell depletion and BMT
Canine model, low-dose TBI combined with BMT and immunosuppression
In vivo T-cell depletion
Miniature swine model, CD3 antibody and CsA
[34]
Co-stimulation blockers
Nonhuman primates model, TBI, CsA, anti-CD154 antibody
[45]
Co-stimulation blockers
Rhezus monkey model, nonmyeloablative conditioning with busulfan, anti-CD40 ligand antibody, sirolimus, anti-CD25 antibody, BMT
[47]
It needs to be emphasized that the animal studies assessing the mechanism of tolerance induction via chimerism are crucial for the transition of this approach toward clinical applications.
Scientific efforts and experimental protocols of Billingham, Ilstadt, Sachs, and many other investigators studying tolerance induction in animal models opened a new era in the field of solid organ and VCA transplantation. As a natural follow-up to these experimental studies, clinical trials on tolerance induction were introduced. The first reports referring to chimerism were based on bone marrow transplantation (BMT). In the late 1970s, reports confirmed that donor-specific blood transfusion in humans often led to the improved allograft acceptance in kidney transplant recipients [75, 76, 93, 119]. Consequently, studies on mice [65], dogs [15, 61, 29], and monkeys [113, 114] showed that the infusion of the donor BMCs combined with the depletion of recipient T cells prolonged allograft survival without the need for toxic and chronic immunosuppression . The first clinical attempt to use donor BMCs in combination with polyclonal antilymphocyte globulin therapy was performed by Monaco et al. in kidney transplant patients [66]. The use of BMT was performed as a supportive cellular therapy in solid organ transplants. Initially, piloted in only a few patients, bone marrow grafts (11 × 109 BMCs) were given 21–25 days after kidney transplantation in combination with immunosuppressive protocol consisting of: azathioprine, antilymphocyte globulin, and prednisone [66]. The early results were encouraging. Decreased levels of kidney graft rejection and decreasing levels of donor responsiveness were observed [1]. In another study, post-kidney transplant administration of donor BMCs (2–3 × 108 BMCs/kg) was performed and supported by cyclosporine A (CsA), prednisone, azathioprine, and antilymphocyte globulin immunosuppressive protocol [61]. Although not randomized, improved kidney graft survival was observed at both 12 and 18 months in the group that received donor BMCs [6]. However, no differences were observed in renal graft function or in the rejection episodes between the two groups [1, 31]. In 1997, the first randomized trial was performed in liver transplant patients and supported by perioperative BMT showing significantly better results in BMT cases, thus favoring the protocol of multiple donor bone marrow infusions. Specifically, both patient and liver graft survival were greater in patients who received multiple bone marrow infusions than the controls who did not receive bone marrow or who received a bone marrow infusion only on the day of liver transplant. In addition, this study confirmed that cytoablative conditioning was not necessary to improve allograft survival when the recipient was given multiple bone marrow infusions [1, 86]. Recently, another study on kidney transplantation was performed following total lymphoid irradiation, antithymocyte globulin, CsA, prednisone, and mycophenolate mofetil (MMF) followed by the administration of 1 × 106 CD3+ T cells and 8 × 106 CD34+-enriched hematopoietic cells/kg [94]. Impressively, this regimen established mixed chimerism and tolerance toward the kidney allograft to such an extent that all immunosuppressive medications were discontinued at 6 months after transplantation. Subsequently, this protocol led to stable kidney graft function and withdrawal of immunosuppression in eight out of 12 patients [1, 95].
In contrast to the augmentation of chimerism by BMT, donor microchimerism commonly refers to chimerism that is detectable after solid organ transplantation without BMT support. In these cases, microchimerism is a consequence of passenger leukocytes migrating out of transplanted tissues. Starzl reported one of the first cases indicating the existence of chimerism after liver transplantation [110, 115]. His group reported systemic microchimerism after a successful liver transplant and suggested that immunosuppression in transplant recipients promotes the microchimeric state. Examples of clinical studies confirmed that the essential condition required for the development of donor chimerism is the migration of passenger leukocytes from transplanted tissue into the host. Confirmation of leukocyte migration was reported in kidney transplants where a large leukocyte population originating from the recipient cells was found [109]. Donor-derived cells were also found in the skin and lymph nodes of the recipients [109]. The presence of donor DNA was confirmed by polymerase chain reaction (PCR) and detection of peripheral blood chimerism. Moreover, donor-specific nonreactivity was confirmed by mixed lymphocyte reaction (MLR) assays. The fact that donor cells were found in the lymphoid organs, peripheral blood, and bone marrow compartments confirms their ability to migrate and be distributed throughout the body. This suggests that a cumulative effect of donor cell engraftment must be considered as a significant phenomenon.
In 1990, Larsen et al. published their observations regarding migration and function of dendritic leukocytes after transplantation [55]. They reported that the infused donor BMCs or passenger leukocytes that migrated out of the transplanted organ in the nonimmunosuppressed murine recipients first circulated in the peripheral blood and then disappeared [63]. Starzl and Elwood observed a similar continuing pattern of BMCs circulation in the posttransplantation patients [24, 91]. The highest concentration of the infused BMCs was recorded in the peripheral blood up to three months following transplantation and then gradually decreased to the minimal levels by one year after cellular therapy infusion [24]. These observations indicate that there are preferential sites for bone marrow-derived cell engraftment as well as differences in cell concentration and localization throughout the recipient body.
Questions thus arise as to how the chimeric cells replicate or prolong their proliferation and long-term survival in the recipient body? What are the immunological mechanisms and functions that allow for the acceptance and existence of the chimeric cells? Based on animal experimental models and experiences in human organ transplantation, the phenomenon of mutual immunosuppression plays an important role in the maintenance of graft survival .
Chimerism-Based Strategies for Tolerance Induction: Cleveland Clinic Experience
Since confirmation of the crucial role of passenger leukocytes by Starzl in his pioneering work, the quest for discovering immunomodulatory properties of chimerism and its correlation with tolerance induction in solid organs and vascularized composite allograft (VCA) transplants is still being investigated [20, 110]. During the last decade, the Siemionow laboratory has been actively involved in the development of tolerance-inducing strategies by introducing new VCA models and performing basic science research on new tolerance inducing immunosuppressive protocols and cell-based therapies.
Siemionow’s team designed a variety of VCA experimental models and evaluated the effects of different tolerance inducing protocols on the development of donor-specific chimerism and VCA transplant survival (Table 16.2). Using the single-tissue (skin) vascularized allotransplantation model, the Siemionow team showed that donor-derived cells present in the graft are migrating to the recipient’s lymphoid compartments and leading to chimerism induction. Furthermore, these studies confirmed the importance of immunosuppressive protocol adjustments for chimerism induction and maintenance.
Table 16.2
Experimental VCA models for chimerism and tolerance induction developed and tested in the Siemionow laboratory
Model | Immunosuppressive/immunodepletive therapy | Supportive cellular therapy | Study conclusion | References |
---|---|---|---|---|
Total abdominal wall transplantation (LBN to Lewis rat) | CsA tapered from 16 mg/kg/day to 2 mg/kg/day over 4-week period | – | Single-tissue component (skin) under adequate immunosuppressive therapy is capable of long-term chimerism induction and extends allograft survival | [70] |
Vascularized and nonvascularized skin allograft transplantationLBN to Lewis rat) | CsA tapered from 16 mg/kg/day to 2 mg/kg/day over 4-week period | – | Development of donor-derived chimerism is affected by the allograft size and vascularization | [71] |
Limb semi-allogenic transplant(LBN to Lewis rat) | Comparison between fluocinolone acetonide (50 mg/ml) applied topically, cyclosporine A 4 mg/kg/day,combined systemic cyclosporine with topical fluocinolone acetonide | Vascularized bone marrow component of the graft | Extended allograft survival was accomplished by combination of low-dose CsA and topical steroids | [38] |
Comparison between ALS only (0.4 mL/kg), CsA only (16 mg/kg), and a combination of CsA and ALS, conditioning was administered 12 h before surgery at three different intervals (7, 14, and 21 days) | Vascularized bone marrow component of the graft | Correlation of donor-specific hematopoietic chimerism with transplant tolerance | [80] | |
Limb allogenic transplant (BN to Lewis rat) | CsA and ALS were administered 2 h before surgery and continued for 21 days. Tapered CsA,16 mg/kg/day,1st week; 8 mg/kg/day, 2nd week; 4 mg/kg/day, 3rd week. ALS—0.4 ml/day for the 1st week, every other day for the 2nd week, and twice weekly during the 3rd week | Vascularized bone marrow component of the graft | Extended survival of the allogenic limb transplant associated with transient chimerism | [78] |
Face/scalp semi-allogenic (LBN to Lewis rat) | CsA 16 mg/kg/day every 24 h after transplantation; tapered to 2 mg/kg/day over 4-week period and maintained at that level thereafter | – | Confirmation of the feasibility of the model with > 170 days survival on maintenance immunosuppression | [117] |
Limb semi-allogenic transplant (LBN to Lewis rat) | 35-day course of 250 μg/kg/day anti-αβTCR antibody and 16 mg/kg/day CsA | Vascularized bone marrow component of the graft | Confirmation of induction of donor-specific tolerance to rat hind-limb allografts (graft survival over XX) | [100] |
Skin allograft (LBN to Lewis rat) | 35-day course of 250 μg/kg/day anti-αβTCR antibody and 16 mg/kg/day CsA | “crude” bone marrow transplantation | Combination of anti-αβTCR antibody and CsA extended skin allograft survival up to 65 days | [105] |
Limb semi-allogenic transplant (LBN to Lewis rat) | 21-, 7-, and 5-day course of 250 μg/kg/day anti-αβTCR antibody and 16 mg/kg/day CsA | Vascularized bone marrow component of the graft | Clinical tolerance and immunocompetence were confirmed by skin grafting in vivo and MLR in vitro. High level of donor chimerism in the peripheral blood of long-term survivors was detected | [79] |
Limb allogenic transplant (BN to Lewis rat) | 7-day protocol of 250 μg/kg/day anti-αβTCR antibody and 16 mg/kg/day CsA | Vascularized bone marrow component of the graft | Seven days combined therapy of anti-αβTCR and CsA induced tolerance in rat hind-limb allografts. Tolerance was directly associated with stable, donor-specific chimerism | [102] |
Hemiface transplantation(LBN to Lewis rat and ACI to Lewis rat) | CsA 16 mg/kg/day, tapered to 2 mg/kg/day and maintained at that level thereafter | – | Survival in 100 % of LBN graft up to 400 days and ACI up to 330 days Low-dose immunosuppression facilitates engraftment of donor-derived cells into the lymphoid organs (lymph nodes and spleen) and supports chimerism induction | [103] |
Hemiface/calvaria transplantation(LBN to Lewis rat) | CsA 16 mg/kg/day tapered to 2 mg/kg per day over 4-week period and maintained thereafter | Vascularized bone marrow component of graft | New reconstructive graft model with survival up to 220 days. Development of donor chimerism—predominantly in the B cell population | [125] |
Maxilla transplantation(LBN to Lewis rat) | CsA 16 mg/kg/day tapered to 2 mg/kg per day over 3-week period and maintained thereafter | Vascularized bone marrow component of graft | Maxilla graft survival up to 105 days. High level of donor-specific chimerism for T cell and B cell lineages was maintained in the peripheral blood | [126] |
Hemiface/mandible/ tongue transplantation (LBN to Lewis rat) | CsA 16 mg/kg/day tapered to 2 mg/kg per day over 4-week period and maintained thereafter | Vascularized bone marrow component of graft | Long-term allograft survival (up to 385 days) correlated with development and maintenance of donor-specific chimerism in lymphoid organs and BM compartment | |
Composite osseomusculocutaneous sternum, ribs, thymus, pectoralis muscles, and skin allotransplantation model (LBN to Lewis rat) | CsA 16 mg/kg/day tapered to 2 mg/kg/day within 4 weeks and maintained thereafter | Vascularized bone marrow component of graft | Long-term allograft survival correlated with development and maintenance of donor-specific chimerism | [12] |
Single-tissue component models provided experience and knowledge that allowed progression to more surgically and immunologically advanced multi-tissue models. In 2000, the Siemionow team was the first to perform a full composite face/scalp allograft transplantation in the rat. The report on the semi-allogenic full-face transplant model in 2003 discussed the role of tapered and low-dose CsA monotherapy on the long-term allograft survival [101]. The development of the less surgically challenging hemiface transplantation model allowed assessment of the immunological responses of face transplant recipients to different immunosuppressive protocols. The study, which compared allogenic and semi-allogenic hemiface transplants, confirmed engraftment of donor-derived cells into lymphoid tissues, such as lymph nodes and spleen in both experimental groups. There was a significant difference between the groups, however, in the level of donor-specific chimerism for both the T and B cell lineages. Following a fully allogenic face transplantation, a higher level of chimerism was observed for both the CD4 and CD8 T cell populations, whereas a significantly reduced chimerism level was found for the B cell lineage when compared with the semi-allogenic hemiface transplants.
The introduction of multi-tissue transplant models containing bone marrow components such as limb, calvaria, maxilla, or hemiface/mandible/tongue transplantation confirmed that the addition of the bone marrow component in combination with adjusted immunosuppressive protocol significantly increased allograft survival (400–700 days). High chimerism levels in these experimental designs encouraged Siemionow’s team to further evaluate the immunomodulatory role of BMT and its potential application as the supportive therapy facilitating allograft acceptance and long-term survival.
Selection of Immunosuppressive Protocol for Chimeric Cell Therapy
According to literature reports, it is confirmed that the highest success rate for extension of allograft survival, chimerism, and tolerance induction is achieved by application of immunosuppressive protocols combining targets which are affecting different stages of the immunologic response [92]. When used as a monotherapy, immunosuppressants, such as immunodepletive agents (Table 16.1), calcineurin inhibitors (CsA, Tacrolimus), mammalian target of rapamycin (mTOR) inhibitors (sirolimus), steroids (methylprednisolone), or co-stimulatory blockade antibodies, are not effective in tolerance induction and have to be used daily in high concentrations. This increases drug-related side effects, morbidity, and mortality. On the other hand, cessation of these immunosuppressive agents leads to acute rejection. Thus, major research centers worldwide are focusing on the development of protocols inducing tolerance and/or chimerism in VCA models by taking advantage of the synergistic effect of combining induction therapy with immunodepletive antibodies, followed by maintenance therapy using calcineurin inhibitors, steroids, and MMF. The immunosuppressive protocols designed for hand transplants include conventional triple therapy, Louisville protocol, Innsbruck protocol, and Pittsburgh protocol; protocols supporting face transplants include conventional triple therapy and Lyon protocol (Table 16.3).
Table 16.3
Immunosuppressive protocols in the clinical cases of hand and face VCA transplantation
Protocol | Induction therapy | Maintenance immunosuppression | Cellular treatment | References |
---|---|---|---|---|
Conventional Hand, face | ATG or alemtuzumab | Triple therapy of corticosteroids, calcineurin inhibitors, and mycophenolate mofetil | N/A | |
Louisville Hand | Alemtuzumab | Tacrolimus and mycophenolate mofetil | N/A | [44] |
Innsbruck Hand | Alemtuzumab | Tacrolimus and prednisone | N/A | |
Pittsburgh Hand | Alemtuzumab | Tacrolimus | Donor-specific bone marrow cell transfusion within 2 weeks after transplantation | [ 97] |
Lyon Face | ATG | Tacrolimus prednisone and mycophenolate mofetil | Donor bone marrow transplantation at day 4 and 11 after transplantation |
Over the past decade, the majority of VCA clinical cases used immunosuppressive protocols originating from kidney transplant experience [17, 81, 96]. However, the dosage of immunosuppressive agents used in clinical VCA is either similar or higher when compared to kidney transplant protocols due to the histological heterogeneity of different tissue components of VCA transplants. Therefore, there is still a great need for the development of new protocols that would facilitate induction of tolerance associated with donor-specific chimerism. The introduction of supportive cellular therapies is a new and exciting option for the prevention of both acute and chronic allograft rejection and the promotion of tolerance induction. Cellular therapies may enhance the development of chimerism and help to induce tolerance secretory functions or through cell-to-cell interactions. It is crucial, however, to adjust current immunosuppressive protocols to the specific types and characteristics of supportive cellular therapies.
The Siemionow laboratory has tested different dosages and timing of the ALS induction therapy, as well as introduced a new immunodepletive agent—the anti-αβ T cell receptor (anti-αβTCR) monoclonal antibody, to the field of VCA. The study of the rat semi-allogenic limb transplantation showed that a 21-day protocol of combined ALS and CsA induced tolerance and significantly prolonged limb allograft survival (over 420 days). Extended survival of the allograft was associated with a high level of donor chimerism measured in the peripheral blood of the recipient. Tolerance was confirmed ex vivo by MLR assay as well as in vivo by the acceptance of the donor skin graft and rejection of the third-party graft (Table 16.2; 80]. Although application of the same immunosuppressive protocol of ALS and CsA in the case of allogenic limb transplantation between BN and Lewis rats extended allograft survival (up to 56 days) and was associated with a transient, low level of chimerism, it is important to note that tolerance was not induced [78].
In the process of optimizing an immunosuppressive protocol to support bone marrow-based therapies such as donor–recipient chimeric cell transplantation, the Siemionow group applied the selectively blocking anti-αβTCR monoclonal antibody induction therapy combined with CsA. The main advantage of using the αβTCR monoclonal antibody is the selective depletion of both the immature and mature alloreactive T lymphocytes responsible for the first signal of T cell activation, when preserving small populations of gamma-delta T cells known for their tolerogenic properties.
An additional advantage of this protocol is decreased expression of intragraft pro-inflammatory cytokines such as interleukin 2 (IL-2) and interferon γ (IFNγ), which are associated with allo-recognition, development of rejection, and endothelial activation. Increased expression of Th2 cytokines (IL-10 and IL-4) suggests that the mechanism of action of this antibody includes a switch from Th1 to Th2 response, allowing for long-term acceptance of the allografts [28]. Additionally, the anti-αβTCR antibody can facilitate the beneficial effects of cellular therapies due to the fact that it does not target cells of the myeloid origin (monocytes, granulocytes) or lymphoid cells such as natural killer (NK) cells, gamma delta (γδ) T cells and B cells. These cells may play a significant role in the development of chimerism and tolerance induction and protect the recipient against potential infections. Induction therapy with the anti-αβTCR antibody was combined with the use of the calcineurin inhibitor CsA. The use of CsA as a monotherapy requires higher dosages, which are toxic and cause a number of serious adverse reactions such as nephrotoxicity and hepatotoxicity. It has been shown that the application of high doses of CsA can reduce the number of interdigitating cells, the size of the thymic medulla, and can also change the morphology of epithelial cells [85]. Thus, high doses of CsA obstructs engraftment of the donor cells and prevents the effective development of a new repertoire of tolerogenic T cells that could facilitate allograft survival by changing the microenvironment in the recipient thymus [85].
Siemionow’s group developed a new clinically applicable nonmyeloablative 35-day protocol of anti-αβTCR monoclonal antibody (250 μg/kg/day) in combination with tapered CsA (16 to 2 mg/kg/day) therapy based on the previous experience with the limb allograft transplantation model under the ALS and CsA immunosuppressive protocol [100]. Experimental animals, which received hind-limb transplants under the anti-αβTCR/CsA protocol, developed tolerance (survival over 720 days) as well as stable donor-specific chimerism observed in the T cell population. Further studies revealed that optimizing the duration of 21, 7, and 5 days of the anti-αβTCR/CsA protocol resulted in long-term limb allograft survival at all time points. Long-term survival of limb allografts was associated with stable chimerism maintenance. The 7-day αβTCR/CsA protocol was chosen to support the future chimeric cell therapy due to the fact that it provides fast immunodepletion of T cells and is sufficient in creating immunological unresponsiveness to the newly introduced donor-derived cells, thus allowing for cell engraftment into the bone marrow niches and lymphoid organs—specifically into the thymus where the interaction with a new repertoire of T lymphocytes may occur and lead to negative selection of the host alloreactive T cells. This protocol is nonmyeloablative and safer for patients by providing protection against bacterial and viral infections. It also provides stable chimerism for both the T and B cell lineages and does not require preconditioning which makes it clinically applicable to cadaveric VCA transplants.
Chimerism as a Base for Development of Chimeric Cell Therapy: Primary and Secondary Chimeric Animals’ Creation
Multiple studies have shown that transplants containing a bone component are more efficacious in chimerism induction and maintenance compared to those without, such as vascularized skin allografts [12, 106]. Vascularized donor bone components provide a continuous source of hematopoietic stem cells that mature, differentiate, and are ultimately responsible for chimerism establishment and preservation in the recipient. In the case of solid organs transplants or VCA that do not contain a bone component, such as face or abdominal wall transplants, bone marrow infusion could be an alternative therapy that supports chimerism induction and increases allograft survival. Billingham et al. demonstrated in their study using neonatal mice that transplantation of hematopoietic cells can induce tolerance of the recipient to the skin allograft via chimerism development [10]. Several animal and clinical case studies confirmed the beneficial effect of bone marrow-derived therapies for survival of the solid organ allografts [8, 46, 49].
To assess the role of hematopoietic cells in chimerism and tolerance induction , the Siemionow group designed a series of experiments in which chimeric animals were created by an adoptive transfer of allogenic (ACI responder to transfused ACI (RT1a)) or semi-allogenic (RT1l+n) BMCs to Lewis rat recipients (RT1l). Animals received a nonmyeloablative 7-day immunosuppressive protocol of anti-αβTCR/CsA on the day of transplantation, and chimerism in both allogenic and semi-allogenic models was successfully developed. Interestingly, Siemionow’s group detected major histocompatibility complex (MHC) antigens specific for both the donor and recipient, ACI (RT1a) donor and Lewis (RT1l) recipient in the allogenic model and LBN (RT1l+n) donor and Lewis (RT1l) recipient in the semi-allogenic model, which were observed on the surface from the isolated recipient (Lewis rat, RT1l) bone marrow cells. To further investigate the properties of these in vivo created donor–recipient (RT1a/RT1l) and (RT1l+n/RT1l) chimeric cells , the Siemionow group harvested BMCs from the primary chimera animals. Next, donor–recipient chimeric cells were isolated using specific monoclonal MHC RT1a and RT1n antibodies and a magnetic-activated cell sorting (MACS) method. Isolated donor–recipient (RT1a/RT1l) and (RT1l+n/RT1l) chimeric cells were used as a supportive therapy for VCA transplantation of allogenic (RT1a) or semi-allogenic (RT1l+n) skin graft to Lewis recipients (RT1l) thereby creating secondary chimeric cells. VCA survival in these animals was up to 365 days post transplant, compared to 84 days in animals receiving no supportive cellular therapy (manuscript in preparation). These results showed that in vivo created donor–recipient chimeric cells carry pro-tolerogenic properties, which significantly improve VCA survival and could be a breakthrough in tolerance induction treatment.
Evaluation of in vivo created donor–recipient chimeric cells in a more complex hemiface transplantation model (manuscript in preparation) confirmed that chimeric cell therapy successfully induces chimerism and increases survival of fully MHC-mismatched hemiface allografts.
Donor–Recipient Chimeric Cells: Potential Mechanism of Chimeric Cell Creation in vivo
The mechanism of the creation of cells, which express markers characteristic of both the donor and recipient, may be explained based on the knowledge gained from stem cell research. There are only a few hypotheses describing the fate of donor-derived cells in the recipient tissues. Following bone marrow infusion, stem cells may undergo different processes, such as differentiation, transdifferentiation, and cell fusion [22], or following cell maturation, they may undergo surface antigen transfer (trogocytosis). Siemionow’s group considers trogocytosis and/or in vivo cell fusion as the most probable mechanism of spontaneous in vivo creation of chimeric cells .
Trogocytosis
Trogocytosis is a widespread phenomenon of the rapid exchange of the membrane antigens between two interacting cells [42]. In 1972, Cone et al. provided the first evidence of trogocytosis (from Greek trogo-, meaning nibble or gnaw [19, 35] when they observed transfer of MHC class II proteins from B to T cells [19]. In the literature, it is described as a transfer of membrane patches containing proteins from the surface of one cell to another following “immune synapse” formation [35]. Trogocytosis is not a protein-selective process. During the transfer, some of the adjacent proteins can also be passively transferred together with the membrane patch. It is regulated by Src kinases and depends on several factors, including adenosine triphosphate (ATP), calcium mobilization, and actin cytoskeleton reorganization [107].
The mechanism leading to trogocytosis in vivo, types of cells involved in the process, and functional consequences are still under investigation. Trogocytosis is currently perceived as a process that can generate complex adaptations in the immune system and results in immune plasticity beyond genetics and epigenetic programming. Acquisition of membrane patches by immune cells may significantly change their phenotype and augment or diminish immune function by generation of activated or immunosuppressive and regulatory cells [25, 56, 57].
Several studies confirmed that lymphocytes, including both B and T cells, are able to acquire or lose proteins relevant for their function [14, 23, 62, 69, 90, 128]. It has also been reported in murine models that CD4- and CD8-positive T cells can acquire APC-derived MHC class I and II molecules and the co-stimulatory molecules B7-1 (CD80), B7-2 (CD86), and I-CAM1 (CD54; 33, 35, 36, 87, 111, 123]. It has been demonstrated that human NK cells can acquire MHC class I or II protein and viral receptors from the target cells [68, 69, 89]. An in vitro study performed by Huang et al. showed that cytotoxic T lymphocytes (CTLs) after trogocytosis of MHC molecules loaded with antigenic peptide recognized by their own T cell receptor, can be eliminated from the organism through cytolysis (called “fratricide”; [33]). Trogocytosis observed in murine models is unidirectional, which means that proteins are transferred exclusively from the recipient to donor cells [16]. In the human experimental model, however, the process of trogocytosis is bidirectional. The phenomenon of intercellular membrane transfer is currently being tested as a tool for engineering immune cells presenting preferential membrane protein patterns for therapeutic application and could be used in the future for adoptive immunotherapy.
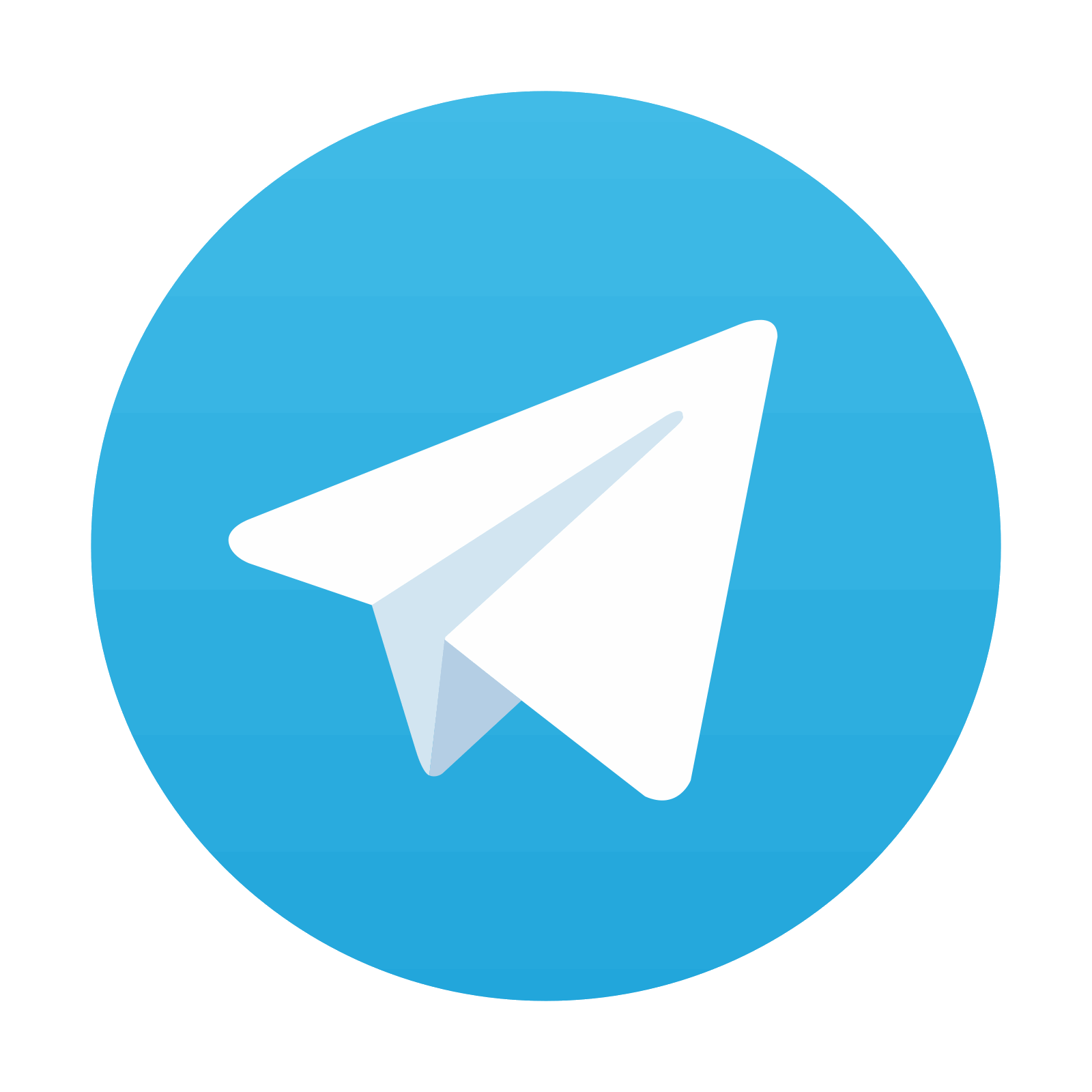
Stay updated, free articles. Join our Telegram channel
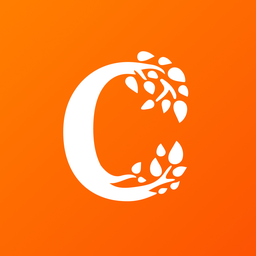
Full access? Get Clinical Tree
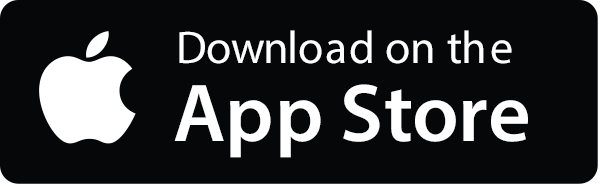
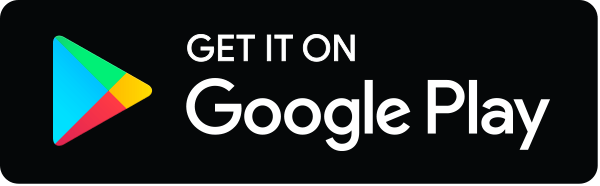