Chapter 14 Anesthesia for burned patients
Access the complete reference list online at http://www.expertconsult.com
IN THIS CHAPTER
PowerPoint Presentation Online
Introduction
Continuous improvements in burn care since World War II have resulted in a steady increase in the rate of survival after large burn injury.1 These improvements have been attributed to aggressive fluid resuscitation, early excision and grafting of burn wounds, more effective antimicrobials, advances in nutritional support, and development of burn centers. The development of specialized burn centers has allowed the concentration and coordination of resources needed to provide a multidisciplinary approach from the time of admission, with the goal of not just maximizing survival but of optimizing functional recovery as well.
Today, most patients who are in good health prior to injury will survive a more than 80% total body surface area (TBSA) burn if promptly treated in a modern burn unit. In their study of risk factors for death following burn injuries Ryan et al.2 identified three variables that can be used to estimate the probability of death: age over 60 years, burns over more than 40% of TBSA, and the presence of inhalation injury. Mortality increased in proportion to the number of risk factors present: 0.3%, 3%, 33%, or approximately 90% mortality depending on whether zero, one, two, or three risk factors were present, respectively. O’Keefe et al. observed an approximately twofold higher risk of death in women aged 30–59 years compared to men with similar burns and age.3 Although it has been assumed that very young children are also at increased risk of death from burn injuries, Sheridan et al. found very low rates of mortality in children younger than 48 months who had suffered large burns.4 An increased risk of mortality has been consistently observed in elderly burn patients. All organ systems are influenced by age-related factors of variable expression that can reduce functional reserve. However, aged patients represent a heterogeneous group in terms of physiological reserve, with wide variation in the presence of coexisting diseases and the difference between chronological and biological age. Issues relating to the treatment of burns in elderly patients have recently been reviewed by Keck and colleagues.5 Most studies of mortality associated with burns focus on patient condition prior to burn center admission. Wang and colleagues examined the association of features related to treatment and hospital course with mortality among patients who had experienced massive burns (>70% TBSA).6 In their series, only sepsis, ventilator dependency and reduced platelet count were independent predictors of mortality.
Major burn injury results in pathophysiological changes in virtually all organ systems. Table 14.1 lists and Fig. 14.1 illustrates some of the challenges presented by the acutely burned patient during the perioperative period. In addition to the predictable challenges relating to airway management, monitoring, and vascular access, patient positioning requires close communication and teamwork. Burns involving posterior areas may require turning the patient to the prone position for optimal access (Fig. 14.1). Vascular catheters and endotracheal tubes must be secured with confidence and due care given these lifelines during patient turning.
Table 14.1 Perioperative challenges in the acute burn patient
The current standard of surgical treatment calls for early excision and grafting of non-viable burn wounds, which may harbor pathogens and produce inflammatory mediators with systemic effects resulting in cardiopulmonary compromise. After an extensive burn injury, the systemic effects of inflammatory mediators on metabolism and cardiopulmonary function reduce physiological reserve, and the patient’s tolerance to the stress of surgery deteriorates with time. Assuming adequate resuscitation, extensive surgery is best tolerated soon after injury when the patient is most fit. Another advantage of early excision is that blood loss is less when wound excision is performed soon after the initial burn.7 However, it must be recognized that the initial resuscitation of patients with large burns results in large fluid shifts and may be associated with hemodynamic instability and respiratory insufficiency. Reynolds et al.8 reported that more than half of deaths after burn injuries occur due to failed resuscitation. Some burn patients develop refractory burn shock soon after injury and cannot be resuscitated.8 An important part of the preoperative evaluation is an assessment of the quality of the resuscitation to be sure the patient is adequately prepared to withstand the stress of surgery.
Since the last edition of this book was published, Fuzaylov and Fidkowski have reviewed the anesthetic management of pediatric patients with major burn injuries.9 Patients suffering burn injuries also require surgical treatment for years after the initial injury in order to correct functional and cosmetic sequelae, and anesthetic management for reconstructive burn surgery presents many special problems.10 This chapter, however, will concentrate on the care of acute burn patients. The acute phase of burn injury is defined as the period from injury until the wounds have been excised, grafted, and healed.
Preoperative evaluation
In addition to the standard features of a preoperative evaluation, the anesthetist should focus on certain burn injury-related features that are associated with increased risk and technical challenge when planning perioperative care of the patient with acute burn injuries (Table 14.2). Because physiological changes associated with the burn injury and their resolution during healing are dynamic, the evaluation of the acutely burned patient requires knowledge of the continuum of these changes after the initial injury. The preoperative evaluation must also be performed within the context of the planned operative procedure, which will depend on the location, extent, and depth of burn wounds, time after injury, presence of infection, and existence of suitable donor sites for autografting. It is important to know the mechanism of injury, as this determines the quality of burn injuries and the kinds of associated disorder the patient may present with. As an example, a person burned in the enclosed space of a house and a child who has suffered a scald burn would present with very different associated injuries. As resuscitation and the inflammatory response to injury are dynamic, it is also important to know the time elapsed since injury.
Table 14.2 Major preoperative concerns in acutely burned patients
Initial evaluation of the burn injury
Destruction of skin by thermal injury disrupts the vital functions of the largest organ in the body. The skin provides several essential protective and homeostatic functions (Table 14.3). Treatment of patients with burn injuries must compensate for loss of these functions until the wounds are covered and healed. As a barrier to evaporation of water, the skin helps maintain fluid and electrolyte balance. Heat loss through evaporation and loss of vasomotor regulation in burned skin diminishes effective temperature regulation. The skin’s barrier function also protects against infection by invading organisms. Wound exudate rich in protein depletes plasma proteins when large body surface areas are injured.
Adapted from Williams WG, Phillips LG. Pathophysiology of the burn wound. In: Herndon DN, ed. Total burn care, 1st edn. London: WB Saunders; 1996: 64.
Much of the morbidity and mortality associated with burn injuries are related to the size of the injury. The extent of the burn injury is expressed as the total body surface area (TBSA) burned. Estimates of TBSA burned are used to guide fluid and electrolyte therapy and to predict surgical blood loss. Initial estimates of the percentage of the skin’s surface that has been burned can be quickly estimated by the so-called ‘Rule of Nines;’ estimates are modified for pediatric patients because of age-related differences in body proportions. More precise estimates of TBSA affected can be made using a Lund–Browder chart (Fig. 14.2).
With extensive wound excision or debridement large amounts of blood can be lost rapidly. Adequate preparation in terms of monitors, vascular access, and availability of blood products is essential. Accurate estimates of blood loss are crucial in planning preoperative management of burn patients. Surgical blood loss depends on the area to be excised (cm2), time since injury, surgical plan (tangential vs fascial excision), and the presence of infection.7 Blood loss from skin graft donor sites will also vary depending on whether it is an initial or a repeat harvest. These variables are valuable predictors of surgical blood loss, which is a critical factor in planning anesthetic management (Table 14.4).
Table 14.4 Calculation of expected blood loss
Surgical procedure | Predicted blood loss |
---|---|
<24 h since burn injury | 0.45 mL/cm2 burn area |
1–3 days since burn injury | 0.65 mL/cm2 burn area |
2–16 days since burn injury | 0.75 mL/cm2 burn area |
>16 days since burn injury | 0.5–0.75 mL/cm2 burn area |
Infected wounds | 1–1.25 mL/cm2 burn area |
Adapted from Desai et al. Early burn wound excision significantly reduces blood loss. Ann Surg 1990; 221: 753–762.10
Airway and pulmonary function
Special attention must be paid to the airway and pulmonary function during preoperative evaluation. In the emergency department burn patients may initially present with respiratory issues that demand immediate attention. In addition, there is general recognition that smoke inhalation injury increases morbidity and mortality for burn patients.11 The presence of an inhalation injury in combination with a cutaneous burn increases the volume of fluid required for resuscitation by as much as 44%, and this may contribute to the increased hemodynamic instability seen in patients with combined injury.12 Numerous studies have also shown an increased incidence of pulmonary complications (pneumonia, respiratory failure, or ARDS) in patients with burns and inhalation injury compared to burns alone.13 Sequelae of inhalation injury include upper airway distortion and obstruction from direct thermal injury, impaired pulmonary gas exchange due to effects of irritant gases on lower airways and pulmonary parenchyma, and systemic effects of inhaled toxins such as carbon monoxide (CO) or cyanide. These three components of the inhalation injury have separate time courses and pathophysiological consequences.
Foley described findings of 335 autopsies performed on patients who died from extensive burns.14 Intraoral, palatal, and laryngeal burns were not uncommon among patients with inhalation injuries. The most common sites of laryngeal injury were the epiglottis and vocal folds where their edges were exposed. In contrast, thermal necrosis below the glottis and upper trachea was not observed in any of these patients. The lower airways are nearly always protected from direct thermal injury by the efficiency of heat exchange in the oro- and nasopharynx, unless the injury involves steam or an explosive blast. This has been demonstrated in an experimental model.15 Inhalation injury to the lower airways and pulmonary parenchyma is due to the effect of toxic or irritant gases.
Clinical suspicion of inhalation injury is aroused by the presence of certain risk factors, such as history of exposure to fire and smoke in an enclosed space, or a period of unconsciousness at the accident scene, burns including the face and neck, singed facial or nasal hair, altered voice, dysphagia, oral and/or nasal soot deposits, or carbonaceous sputum. The most immediate threat from inhalation injury is upper airway obstruction due to edema. Early or prophylactic intubation is recommended when this complication threatens. However, exposure to smoke does not always lead to severe injury, and in the absence of overt evidence of respiratory distress or failure it may be difficult to identify patients who will experience progressive inflammation and ultimately require intubation of the trachea. In a retrospective study, Clark et al. reported that 51% of their patients exposed to smoke inhalation did not require intubation.16 Unnecessary intubation in the presence of an inflamed laryngeal mucosa risks further damage to the larynx and subglottic area.17,18
Traditional clinical predictors of airway obstruction have been found to be relatively insensitive and inadequate for identifying early severe airway inflammation, and often underestimate the severity of the injury.19,20 More objective criteria for evaluation of the risk of airway obstruction are often needed. Hunt et al. found fiberoptic bronchoscopy to be a safe and accurate method for diagnosis of acute inhalation injury.21 They described observations of severe supraglottic injuries associated with mucosal edema obliterating the piriform sinuses and causing massive enlargement of the epiglottis and arytenoid eminence. Haponic et al. made serial observations by nasopharyngoscopy in patients at risk for inhalation injury and found distortions of the upper airway described as compliant, edematous mucosa of the aryepiglottic folds, and arytenoid eminences that prolapsed to occlude the airway on inspiration.22 Progressive upper airway edema in these patients was correlated with body surface area burned, resuscitative volume administered, and rate of infusion of resuscitative fluids. Traditional teaching with the support of the American Burn Association has encouraged early prophylactic endotracheal intubation when risk factors for upper airway inhalation injury are present. In response to what appeared to be a preventable death associated with a prehospital intubation, Eastman and colleagues at the Parkland Burn Center performed a retrospective study to identify the factors that led to prehospital intubation of burn victims and, by examining the patients’ hospital courses, to determine which intubations were indicated.23 Of 879 patients intubated prior to hospitalization 11.9% were extubated the day of admission and 41.4% were extubated successfully within 48 hours of injury. This implies that many patients were intubated without benefit. The authors suggested that improved training of prehospital providers might reduce the number of unnecessary prehospital intubations. Over the past two decades we have observed serious morbidity and some deaths due to airway complications in patients intubated for transport (unpublished observations). Tracheal intubation is not a benign intervention and should be employed only when there is a clear risk–benefit advantage, especially in the context of transport between facilities. For patients who are at risk for inhalation injury but lack definitive indications for intubation, fiberoptic nasopharyngoscopy is effective in identifying laryngeal edema. Serial examinations may help avoid unnecessary intubations and at the same time identify progressive inflammatory changes and allow intubation before severe airway obstruction and emergency conditions develop.17
The level of respiratory support must also be assessed. The level of required support may range from supplemental blow-by or mask oxygen to intubation and ventilation with high positive end-expiratory pressure (PEEP) and FIO2. Acute lung injury can occur from inhalation of chemical irritants, systemic inflammation from burn wounds or difficulties with resuscitation, or ventilator-induced injury. Common pathologies include upper thermal airway injury with stridor, pulmonary parenchyma damage from chemical irritants or inflammation, lower airway obstruction from mucus plugs and epithelial casts, as well as pulmonary edema due to acute lung injury or volume overload. Lower airway and parenchymal injuries develop more slowly than upper airway obstruction. Prior to resuscitation, clinical signs and symptoms, chest X-ray, and blood gas analysis may be within normal limits despite significant injury that eventually progresses to respiratory failure requiring intubation and mechanical ventilation.24
Linares et al. studied the sequence of morphological changes following smoke inhalation in an experimental sheep model.25 They observed four discrete but overlapping phases of injury, described as exudative, degenerative, proliferative, and reparative. During the first 48 hours the exudative phase was characterized by polymorphonuclear (PMN) infiltration, interstitial edema, loss of type I pneumocytes, and damage to the tracheobronchial epithelium in the form of focal necrosis, hemorrhage, and submucosal edema. The degenerative phase occurred between 12 and 72 hours and was characterized by progressive epithelial damage with shedding of necrotic tissue and the formation of pseudomembranes and casts. Hyaline membranes developed over alveolar surfaces. Macrophages began to accumulate to begin absorption of necrotic debris. A proliferative phase was described between days 2 and 7 during which type II pneumocytes and macrophages proliferated. After the fourth day reparative changes were observed, with regeneration of epithelium from spared epithelium from the orifices of glands.
Demling and Chen have provided a lucid description of the pathophysiological changes following inhalation injury.26 Decreased dynamic compliance increases the work of breathing. Increased closing volume and decreased functional residual capacity lead to atelectasis and shunt, resulting in hypoxia. Airways become plugged by sloughed epithelium, casts, and mucus. Impaired ciliary action exacerbates the airway obstruction by reducing the clearance of airway debris. These changes lead to further shunting and allow colonization and pneumonia. Treatment for inhalation injury is empiric and supportive, with tracheal intubation and mechanical ventilation. The application of aggressive pulmonary toilet, high-frequency percussive ventilation, and respiratory therapy protocols designed to mobilize obstructing debris have been found to be beneficial.27,28 The importance of strategies to limit ventilator-induced lung injury has been recognized.29,30
Carbon monoxide (CO) and cyanide are two major toxic components of smoke. The burn patient with evidence of inhalation injury should be evaluated for the presence of toxicity resulting from these compounds. CO binds hemoglobin 200 times more avidly than oxygen.31 Therefore, CO markedly impairs the association of oxygen with hemoglobin and reduces oxygen-carrying capacity. CO also shifts the oxyhemoglobin dissociation curve to the left, thereby reducing the release of oxygen into tissues. These factors result in decreased oxygen delivery to tissues and, at critical levels, lead to anaerobic metabolism and metabolic acidosis. Signs and symptoms of CO poisoning include headache, mental status changes, dyspnea, nausea, weakness, and tachycardia. Patients suffering CO poisoning have a normal PaO2 and oxygen saturation by routine pulse oximetry. They are not cyanotic. Carboxyhemoglobin must be detected by CO oximetry. Carboxyhemoglobin levels above 15% are toxic and those above 50% are often lethal. The major treatment approach is administration of 100% oxygen. Hyperbaric oxygen has also been used to treat acute CO poisoning, but there is no general agreement on indications, treatment parameters, or outcome benefits. The data have been reviewed recently by Kealey.32 As a result, available data provide no reliable evidence that hyperbaric oxygen reduces the incidence of neurological sequelae of CO toxicity.
Cyanide is also a component of smoke, resulting from the burning of certain plastic products.33 Cyanide directly impairs the oxidative apparatus in mitochondria and reduces the ability of cells to utilize oxygen in metabolism. These alterations result in conversion to anaerobic metabolism and the development of metabolic acidosis. Signs and symptoms include headache, mental status changes, nausea, lethargy, and weakness. Hydrogen cyanide levels above 100 ppm are generally fatal.34,35
Treatment of cyanide toxicity begins with a high inspired oxygen concentration, which may increase intracellular oxygen tension sufficiently to cause non-enzymatic oxidation of reduced cytochromes, or displace cytochrome oxidase and potentiate the effects of administered antidotes. Pharmacological intervention includes methemoglobin generators such as the nitrates (amyl nitrite inhalation 0.2 mL, or sodium nitrite IV 10 mL of 3% solution for adults and 0.13–0.33 mL/kg of 3% solution for children) and dimethylaminophenol (3.25 mg/kg) to increase methemoglobin levels. Methemoglobin competes with cytochrome oxidase for cyanide. However, excessive levels of methemoglobin lead to reduced oxygen-carrying capacity and may be toxic. Direct binding agents have a high affinity for cyanide. Di-cobalt edetate (20 mL of 15% solution for adults or 0.3–0.5 mL/kg of 15% solution for children) is extremely rapid in action but has significant toxicity, whereas hydroxocobalamin (adults 5–10 g or children 70 mg/kg), the precursor of vitamin B12, has been shown to be safe, with few systemic side effects; it is actively metabolized by the liver and avoids renal absorption. Sulfur donors such as sodium thiosulfate (adults 25 mL of 50% solution or children 1.65 mL/kg of 25 % solution) accentuate the body’s enzymatic conversion of cyanide to thiocyanate in the presence of the mitochondrial enzyme rhodanese, reducing its toxicity and increasing elimination.35,36
Effect of burn injury on circulation
After massive thermal injury, a state of burn shock develops due to intravascular hypovolemia and, in some cases, myocardial depression. This state of burn shock is characterized by decreased cardiac output, increased systemic vascular resistance, and tissue hypoperfusion.37,38 Intravascular hypovolemia results from alterations in the microcirculation in both burned and unburned tissues, leading to the development of massive interstitial fluid accumulation. Cutaneous lymph flow increases dramatically in the immediate post-burn period and remains elevated for approximately 48 hours.39 The forces responsible for this massive fluid shift involve components of the Starling equilibrium,40
The specific alterations include:
• an increased microvascular permeability coefficient (kf and σ) due primarily to the release of local and systemic inflammatory mediators;
• an increase in capillary hydrostatic pressure (Pc) due to microvascular dilatation;
• decreased interstitial hydrostatic pressure (Pif) due to disruption in collagen binding;41
• decreased intravascular oncotic pressure (πc) due to leakage of protein from the intravascular space; and
• a relative increase in interstitial oncotic pressure due to a smaller decrease in interstitial oncotic pressure (πi) compared to πc.
Burn injury is unique with regard to microcirculatory dysfunction. Perturbations occur in all of the Starling forces. Marked edema formation in burn-injured skin occurs almost instantaneously owing to a large negative interstitial hydrostatic pressure.42 Other hydraulic forces coupled with microvascular permeability lead to the leakage of protein and fluid into the interstitial space. Although fluid replacement therapy is essential in treating hypovolemia, edema is worsened by plasma protein dilution (reduction in plasma oncotic pressure). The net effect of these changes is the development of massive edema during the first 24–48 hours after thermal injury and a concomitant loss of intravascular volume.
The hypotension associated with burn injury could also be due to myocardial depression.43–47 Thermal injury stimulates the release of inflammatory mediators such as tumor necrosis factor α (TNFα), interleukin-1 (IL-1), and prostaglandins. TNFα and IL-1 are known to have myocardial suppressant effects.48,49 Experimental animal models clearly demonstrate cardiac depression.43–46 Clinical evidence of myocardial depression is less characterized. Measurements of cardiac function after burn injury often differ with respect to time of injury, severity of injury, patient comorbidities, monitor type, e.g. PA catheter, echocardiography, and the sample size of the study.50–56 At our institution, we routinely perform transesophageal echocardiography in severely injured patients within the first 72 hours of admission. Our observational data show that a significant percentage of patients have severe systolic dysfunction, which is associated with a prolonged ICU course (Fig. 14.3).57
If the patient survives the initial burn shock and is adequately resuscitated, an inflammation induced hyperdynamic circulation develops. This state of massive inflammation or systemic inflammatory response syndrome (SIRS) is characterized by hypotension, tachycardia, a marked decrease in systemic vascular resistance, and increased cardiac output. SIRS has a continuum of severity ranging from the presence of tachycardia, tachypnea, fever, and leukocytosis to refractory hypotension and multiple organ system dysfunction. Several fluid resuscitation protocols that use various combinations of crystalloids, colloids, and hypertonic fluids have been developed (Table 14.5). Isotonic crystalloid is the most commonly used fluid for resuscitation in US burn centers. The most popular fluid resuscitation regimen, the Parkland formula, uses isotonic crystalloid solutions and estimates the fluid requirements in the first 24 hours to be 4 mL/kg/% TBSA burned. However, many burn centers are administering 50% more fluid than the Parkland formula would predict.58 Crystalloid solutions generally provide adequate volume resuscitation, but the large volumes that are needed result in substantial tissue edema and hypoproteinemia. Consequently, complications of over-resuscitation, including abdominal compartment syndrome, pleural effusions, pulmonary edema, fasciotomies and conversion of partial-thickness lesions to full-thickness lesions are more frequently observed. Explanation for this pattern of ‘fluid creep’ is unclear.59,60 Clinical practice has changed in the last 30 years. Patients are receiving higher amounts of opioids to treat pain associated with thermal injury, which can cause vasodilation and precipitate hypotension. Additionally, most clinicians appreciate the dangers of hypovolemia; however, there is limited education about the risks of administering too much fluid. Thus, reliance on targeted or goal directed endpoints for fluid resuscitation following severe thermal injury is rarely practiced.
Resuscitation regimens that restore vascular volume losses while reducing edema formation are continuously being tested. Colloid and hypertonic fluids have shown efficacy in treating other injuries. Overall, colloid resuscitation within the first 24 hours of burn injury has not improved outcome compared to crystalloid resuscitation.61,62 Furthermore, a recent meta-analysis indicated that mortality is higher in burned patients receiving albumin as part of the initial resuscitation protocol, with a 2.4 relative risk of mortality compared to patients receiving crystalloid alone.63 Because of the added cost and the little established benefit, colloid solutions have not been used routinely in the United States for initial volume resuscitation in burned patients. However, specific types of colloid regimen might yield different results. Recently, in a prospective, randomized study, the use of plasma for volume resuscitation was shown to limit the volume infused along with reducing intra-abdominal pressure and abdominal compartment syndrome (see below).64 These outcome variables have not been used for comparing crystalloid and colloid resuscitation in the past. With the trend toward larger volumes for initial resuscitation it may be that the use of colloid may be beneficial for larger injuries requiring more volume.
The use of hypertonic saline, either alone or in conjunction with colloids, has been advocated by some in the initial resuscitation of burned patients. Among the potential benefits are reduced volume requirements to attain similar levels of intravascular resuscitation and tissue perfusion compared to isotonic fluids.65 Theoretically, the reduced volume requirements would reduce the incidence of pulmonary and peripheral edema, thereby reducing the incidence of pulmonary complications and the need for escharotomy. Hypertonic saline dextran solutions have been shown to expand intravascular volume by mobilizing fluids from intracellular and interstitial fluid compartments. Although hypertonic saline dextran solutions transiently reduce initial fluid requirements, there is a potential for a rebound in fluid needs later in resuscitation owing to the sodium load.66,67 Therefore, most burn centers continue to use isotonic crystalloid fluids for initial resuscitation of patients in burn shock.
Monitors for resuscitation
Several parameters have been used to assess the adequacy of volume resuscitation in burned patients (Table 14.6). Unfortunately, there is no single physiological variable that is always reliable as an endpoint to guide resuscitation in acute burn patients. The overall goal is early volume resuscitation and establishment of tissue perfusion. Traditionally, urine output (0.5–1 mL/kg/h) and normalization of blood pressure (mean arterial blood pressure > 70 mmHg) have been used as endpoints. However, these parameters alone do not always indicate adequate tissue perfusion.68–70 Jeng and colleagues showed that attaining urine outputs > 30 mL/h and mean blood pressures > 70 mmHg correlated poorly with other global indicators of tissue perfusion, such as base deficit and blood lactate levels.68 During shock, perfusion of vital organs such as the heart and brain is maintained due to the redistribution of blood flow from the splanchnic circulation and other peripheral organs. Persistent hypoperfusion of these organ systems ultimately results in tissue injury and contributes to multisystem organ dysfunction. Therefore, the anesthesiologist should not base the cardiovascular assessment strictly on vital signs and urinary output.
Table 14.6 Criteria for adequate fluid resuscitation
Invasive cardiovascular monitors are not used routinely in burned patients to guide volume resuscitation. Most patients can be adequately resuscitated without their use. However, a small subset of patients, such as those with underlying cardiovascular disease or those who do not respond normally to volume resuscitation – termed non-responders, may benefit from invasive monitoring. Some investigations use cardiac index and oxygen delivery to guide volume resuscitation.71,72 One way in which shock can be defined is oxygen debt. Bernard and colleagues have shown that patients surviving large burn injuries had higher cardiac indices and more effective oxygen delivery than non-survivors.73 Some investigators have proposed the use of supranormal oxygen delivery as a means of assuring adequate tissue perfusion.74,75 The preselected goals were a cardiac index of 4.5 L/m2 and an oxygen delivery index of 600 mL/min/m2. These values represent approximately 150% of normal cardiac index and oxygen delivery values. Attaining supraphysiological cardiac output and oxygen delivery has been shown to improve outcome in some studies. Schiller and colleagues demonstrated that maintaining a hyperdynamic hemodynamic state using fluids and inotropes improved survival in burn patients.76 However, other investigations, including a meta-analysis, have shown that achieving supraphysiological levels of cardiac output and oxygen delivery did not improve mortality or reduce the incidence of organ failure in trauma and burn patients.77–79 The use of inotropes to attain supraphysiological oxygen transport could be detrimental in some cases. One study that used dobutamine to increase cardiac output and increase oxygen delivery demonstrated increased mortality.80 Dobutamine, like other inotropes, can increase cardiac work and result in myocardial ischemia or myocardial infarction. Selective use of inotropes is warranted. In general, our practice is to use inotropic support in patients with dilated cardiomyopathy and withhold it in patients with risk factors for coronary artery disease. Estimating preload in the acutely burned patient is quite challenging. Filling pressures (central venous pressure and pulmonary artery occlusion pressure) correlate poorly with circulating blood volume, especially during positive-pressure ventilation.81 Newer techniques estimate the volume of blood in the thorax (intrathoracic blood volume, or ITBV) using transcardiopulmonary thermodilution.82,83 This technique has been used in burn patients during resuscitation. Holm and colleagues observed that ITBV, but not central venous and pulmonary capillary occlusive pressure, correlated with cardiac index and oxygen delivery during fluid resuscitation of burn patients.83 The use of ITBV to successfully restore cardiac index in this series was associated with significantly larger volumes than predicted by the Parkland formula.83 This technology is available commercially as the PiCCO system (Pulsion Medical Systems, Munich, Germany). In addition to ITBV this system also provides an estimate of extravascular lung water and stroke volume variability, and a continuous estimate of cardiac output and systemic vascular resistance.
Blood lactate and base deficit provide indirect metabolic global indices of tissue perfusion. Lactic acid is a byproduct of anaerobic metabolism and is an indicator of either inadequate oxygen delivery or impaired oxygen utilization. In the absence of conditions such as cyanide poisoning or sepsis that alter oxygen utilization at the cellular level, lactate production serves as a useful marker of oxygen availability. Serum lactate levels have served as a useful marker of fluid resuscitation and tissue perfusion in burn patients.84 A recent study showed serum lactate to be highly predictive of adequate tissue perfusion. A serum lactate level <2 mmol/L in the first 24–72 hours after burn injury correlated with increased survival.85 Base deficit is another indirect indicator of global tissue perfusion. The base deficit is calculated from the arterial blood gas using the Astrup and Siggard–Anderson nomograms. Although it is not directly measured, base deficit provides a readily obtained and widely available indicator of tissue acidosis and shock. Base deficit has been shown to correlate closely with blood lactate and provides a useful indicator of inadequate oxygen delivery. A retrospective study by Kaups et al. showed that base deficit was an accurate predictor of fluid requirements, burn size, and mortality rate.86
Lactate and base deficit serve as global markers of tissue perfusion and oxygen delivery. However, in burn patients tissue perfusion is not uniform. Perfusion of the splanchnic beds is often sacrificed in order to maintain the perfusion of heart, brain, and kidneys. The use of gastric intramucosal pH (pHi) has been advocated as a measure of splanchnic perfusion. Several studies have shown that measurement of pHi is useful in guiding resuscitation, and that low pHi is a predictor of organ failure and death.87 pHi is measured by gastric tonometry and can provide useful information regarding tissue perfusion.
Formulae for resuscitation of burns provide an approximation of fluid needs, but volumes actually administered need to be individualized for each patient. Although two patients may each have 50% TBSA burns, it is not likely that their physiologic responses will be equivalent. Several factors can increase fluid requirements (Table 14.7). Deep full-thickness burns require larger volume than partial-thickness injuries. Likewise, extensive soft tissue damage from electrical burns or crush injuries increases fluid needs. Inhalation injury can also increase fluid requirements as much as 50%, as noted above.12 These differences in wounds and fluid requirements between patients make it very difficult at times to optimize fluid administration. Because no single physiologic endpoint is always reliable, all available clinical information must be examined and each variable evaluated within the context of all the other variables.
Table 14.7 Factors that may increase fluid needs for resuscitation of patients with acute burn injuries
As discussed, increased volumes of crystalloid solutions are being used for resuscitation of burn patients.88 In many cases as much as twice the volume recommended by the Parkland formula is used. Edema is commensurate with volume infused. During the preoperative evaluation attention must be paid to the degree to which edema produces physiologic derangements. The edema can lead to compartment syndrome of extremities or abdomen (Fig. 14.4). Blindness due to ischemic optic neuropathy has been reported as a complication of burn resuscitation.89 Increased intra-abdominal pressure is a complication of vigorous fluid resuscitation, which may be more common than generally appreciated and may often explain difficulties with resuscitation. Greenhalgh and Warden first described the association of increased abdominal pressures and compartment syndrome with burn resuscitation.90 Ivy and colleagues prospectively studied 10 adult patients presenting with >20% TBSA burns and found that 70% of them had at least transient intra-abdominal hypertension.91 Two of their patients with >80% TBSA burns developed abdominal compartment syndrome requiring surgical decompression. Several studies since then have described the common occurrence of increased intra-abdominal pressure with large-volume burn resuscitation. Increased intra-abdominal hypertension is termed abdominal compartment syndrome when it is associated with impaired respiration, circulation, and urine output. Mechanical ventilation is impaired by pressure on the diaphragm, circulation is impaired by restricted venous return due to caval compression, and urine output is impaired by compression of renal vessels. When this pattern presents the patient should be examined for elevated intra-abdominal pressure. This can be accomplished by measuring bladder pressure: 50 mL of saline is instilled into the bladder through the Foley catheter and the height of the saline column above the symphysis pubis is measured (1.36 cm H2O = 1 mmHg).92 Conservative treatment of elevated intra-abdominal pressure includes attempts to limit the volume of intravenous fluid needed for resuscitation. The inclusion of plasma with infused fluids has been found to reduce the volume required and was associated with significantly lower intra-abdominal pressures.64 In addition, adequate analgesia and sedation should be achieved. Diuresis with furosemide and muscle relaxants to reduce muscle tone have been used to reduce intra-abdominal pressure. More invasive measures include escharotomies,93 percutaneous peritoneal dialysis catheter drainage,94 and laparotomy.90
Recently several clinicians have reported a specific type of burn injury that has been associated with difficulties with resuscitation.95–97 There has been a dramatic increase in burn injuries from explosions and fires related to methamphetamine production in illicit laboratories. Victims of these accidents present unique challenges for a variety of reasons. Substances used in methamphetamine production include chemicals that are corrosive and toxic (e.g. anhydrous ammonia, hydrochloric acid, red phosphorus, and ephedrine). Other ingredients are flammable (acetone, alcohol, and gasoline) and explosions can coat the victims with all these chemicals. As a result, in addition to the victim’s toxic exposure, contacting incompletely decontaminated victims of these accidents has injured first responders and hospital workers.98,99 In addition to exposures described above, these patients are usually intoxicated with methamphetamine, as demonstrated by positive urine screen, and may have inhaled toxic fumes such as phosphine gas. Santos et al. found the incidence of inhalation injury to be twice as great in victims of methamphetamine-related burns as in age- and burn-matched controls.95 Among their patients requiring intubation for inhalation injury methamphetamine users also required roughly twice as many ventilator days. Clinical studies have consistently observed increased fluid requirements for resuscitation of methamphetamine patients.95,98 For example, Santos et al. found that resuscitation volumes were 1.8 times greater for methamphetamine users with burns than for controls.95 Methamphetamine users with burns also experienced more behavioral problems. These patients are more often agitated and require restraints. Santos et al. reported that all their methamphetamine patients required greater than normal doses of sedatives and displayed what they referred to as ‘withdrawal-type syndrome,’ 95 possibly owing to withdrawal of methamphetamine from chronic users.
Effect of burn injury on renal function
Acute renal failure (ARF) is a relatively common complication following major burn injuries. The incidence of ARF following burn injury has been reported to be as high as 40% and is most dependent on the size and severity of the burn and the presence of inhalation injury.100–103 The development of ARF is a poor prognostic indicator. Jeschke and colleagues reported a mortality of 56% in pediatric burn patients with ARF.104 A recently reported Scandinavian study that analyzed 1380 adult burn patients reported an overall mortality rate of 44.1% in patients with acute renal insufficiency, with a mortality rate of 62.5% in those requiring renal replacement therapy.103 Holm and colleagues observed that ARF could be divided into early and late categories. Early ARF was defined as occurring within 5 days of burn injury.105 The most common apparent causes of early renal injury were hypotension and myoglobinuria. ARF occurring after 5 days of injury was defined as late. Here, sepsis was the most common cause, with a small number of cases resulting from the administration of nephrotoxic drugs. Factors that will reduce the incidence of ARF and, if it occurs, associated mortality, include adequate fluid resuscitation, early wound excision, and prevention of infection.106 Regardless of the cause, it is critical to assess renal function in burn patients in order to develop a comprehensive anesthetic plan. Important areas of analysis include urine output, dialysis dependence, volume status, and electrolyte concentrations; diuretic therapy should also be noted. Scheduled doses of diuretics may need to be continued during the perioperative period to maintain urine output.
Metabolic changes associated with burn injury
Increased metabolic rate is the hallmark metabolic alteration that takes place after thermal injury. The magnitude of hypermetabolism is influenced by the size of the burn wound, medical management and the core body temperature of the patient.107–111 Within the range of 30–70% TBSA burn injury, hypermetabolism tends to be proportionate to the size of the burn wound. With burns beyond this range, the hypermetabolism appears to plateau and only increases in smaller increments as burn size increases.112 Sepsis can also increase the metabolic response, as does the physiologic stress of pain.109,113 It has been observed that modern treatment of burn injuries with early excision and grafting ameliorates the hypermetabolism.114 As mentioned earlier, burn patients increase their metabolic rate in an effort to generate heat according to an increased core temperature threshold set point, which is influenced by the size of the burn (see Thermoregulation in burn patients, below). The recognition of this fact has led to an increased awareness of the importance of the ambient temperature in modulating the hypermetabolism of the burn patient. Using indirect calorimetry in acute patients with major burn injuries that are treated according to current standards, resting energy expenditures that are 110–150% above predicted values are frequently measured.115 Resting energy expenditure typically increases as core body temperature decreases below the new set point.116 Therefore, it is critical to prevent significant decreases in core body temperature in the operating room.
As a result of the hypermetabolic response, the burned patient has an increased O2 consumption along with an increased CO2 production that collectively causes increased minute ventilation and demands a higher respiratory effort.109 The anesthetic care of the acute burned patient must accommodate these changes, and frequently this has to be done in patients with compromised pulmonary function due to burn and inhalation injuries.
According to the hypermetabolism, the caloric needs of the burn patient are also increased. Furthermore, numerous studies have shown that optimized nutritional care can not only ameliorate the burn-associated state of catabolism and immune suppression but can also improve wound healing.107 Oral or enteral feeding is recognized as optimal for the burned patient. Frequently the acute burn patient has to be fed continuously over extended periods. This is not only because of the increased caloric needs but also because of compromised gastric emptying and decreased intestinal motility, which necessitates a slower feeding rate of critically ill patients. If standard guidelines for perioperative fasting are implemented, recurrent operative procedures can significantly impinge on the nutritional needs of the patient and ultimately cause a caloric deficit. To accommodate the nutritional needs of the patient a continuation of duodenal nutrition perioperatively has been advocated. Studies indicate that not only is this procedure safe but it might also provide for a favorable gut oxygen balance.117
At the time of the withdrawal of ventilator support and extubation the metabolic state of the burn patient should be considered. The characteristic catabolic state of major burn injury spares no muscles109,118 and the respiratory muscles are affected. Along with decreased muscle strength there is frequently decreased pulmonary compliance, due not only to the formation of scar tissue and pulmonary interstitial changes but also to increased intra-abdominal content. Burn-associated hepatomegaly along with gastrointestinal retention can significantly impinge on respiratory reserves.119
Severe insulin resistance with hyperglycemia and concurrent hyperinsulinemia is a key feature of the metabolic alterations of burn injury.108,109,113 It is well recognized that critically ill patients with insulin resistance benefit from tight glycemic control in the ICU,120 and these findings have been expanded to the burn patient population.121 During the intraoperative period the question is less studied. Although the benefit of tight intraoperative glycemic control has been documented in other patient populations,122 the risk versus benefit during anesthesia has not been studied specifically in burn patients.
Thermoregulation in burn patients
Maintenance of proper body temperature is an important factor in the care of severely burned patients. The thermoregulatory system is controlled by three major components. These are the afferent system, which senses changes in core body temperature and transmits this information to the brain; the central regulatory mechanisms located primarily in the hypothalamus that process afferent input and initiate responses; and the efferent limb, which mediates specific biological and behavioral responses to changes in core body temperature (Fig. 14.5). Temperature is sensed by Aδ and C fibers present in peripheral tissues such as skin and muscle as well as core tissues such as brain, deep abdominal tissues, and thoracic viscera.123 The vast majority of afferent input arises from the core tissues. Because the skin is in direct contact with the environment, it senses immediate changes in environmental temperature. However, the overall afferent input of the skin and other peripheral tissues is estimated to be only 5–20% of total afferent thermoregulatory input.123 Therefore, loss of skin following a burn injury is not likely to markedly alter overall afferent input. Wallace and colleagues have shown that burn patients perceive changes in ambient temperature as effectively as normal controls.124 This is likely due to the retained ability of burn patients to sense changes in core temperature and transmit this information to the central nervous system. Central control of temperature is a complicated system that is not well understood. The hypothalamus plays an important role in temperature regulation, but the complete mechanism of temperature control is likely to be multifaceted and is an area of intense research. Regardless of the ultimate control mechanisms, temperature control can be divided into three main functions: threshold, gain, and maximum response intensity.
Threshold encompasses a set point at which responses to temperature change are initiated. In normal individuals the threshold range is generally near 36.5–37.5°C. In burn patients, the threshold set point is higher and the increase is proportional to the size of the burn. The work of Caldwell and colleagues predicts that the temperature set point will increase by 0.03°C/% TBSA burn.125 This increase in temperature threshold appears to be due to the hypermetabolic state and the pyrogenic inflammatory mediators such as TNF, IL-1, and IL-6 that are present after thermal injury. The elevated temperature set point can be reduced by administration of indomethacin, which suggests that prostaglandins act as final common mediators of this response.126,127
Gain describes the intensity of response to alterations in temperature. In most cases the gain of thermoregulatory responses is very high, with response intensity increasing from 10% to 90% with only a few tenths of a degree change in core temperature. This response is maintained in most burn patients, resulting in a further increase in metabolic rate.124 Burn patients respond with a brisk increase in heat generation and metabolic rate in response to changes in core body temperature.124 However, work by Shiozaki and colleagues has shown that burn patients who are slow to respond to postoperative hypothermia are at increased risk of mortality.128 The decreased responsiveness may be due, in part, to tissue catabolism, poor nutrition, or sepsis. In addition, the response to relative hypothermia is characterized by increased catecholamine release, tissue catabolism, and hypermetabolism. These responses further stress burn patients and reduce their ability to respond to their primary injury.129
The most important efferent responses to hypothermia are behavioral responses such as gaining shelter, covering up, and seeking a more desirable ambient temperature. In the acute post-burn setting most of these behaviors are impeded by positioning, sedation, and inability to seek a more favorable environment. Therefore, caregivers must be attentive to the patient’s temperature and perception of cold so that measures can be undertaken to optimize the patient’s temperature. Cutaneous vasoconstriction is another important mechanism for preserving heat and core body temperature. In unburned persons a temperature gradient of 2–4°C exists between skin and core tissues. This gradient is maintained by cutaneous vasoconstriction. Without cutaneous vasoconstriction, heat is redistributed from the core compartment to the periphery. This heat is ultimately lost to the environment. Peripheral vasoconstriction minimizes temperature redistribution and acts to maintain core body temperature. This mechanism of heat preservation is lost after cutaneous thermal injury and excision of large areas of skin, particularly if cutaneous tissues are excised down to the fascial level. The loss of skin facilitates the redistribution of core body heat to the periphery and, ultimately, into the environment, which places the burn patient at risk for core hypothermia. Another mechanism of heat loss in burn patients is evaporation. Burn patients can lose as much as 4000 mL/m2 burned/day of fluids through evaporative losses.130 Mechanisms of non-shivering heat production and shivering remain intact in burn patients. However, shivering increases metabolic requirements and is likely deleterious.
The induction of anesthesia results in relative ablation of thermoregulatory mechanisms and puts the patient at further risk for developing hypothermia. Patients under general anesthesia exhibit a markedly decreased threshold for responding to hypothermia (Fig. 14.6). This is particularly important in burn patients, given their high temperature set point and the deleterious effects of further stress responses and hypermetabolism in this patient population. Most anesthetics reduce nonbehavioral responses to hypothermia, such as vasoconstriction, non-shivering thermogenesis, and shivering. Of course, behavioral responses are ablated during general anesthesia. Therefore, it is the responsibility of the intraoperative caregivers to monitor and maintain patient temperature.
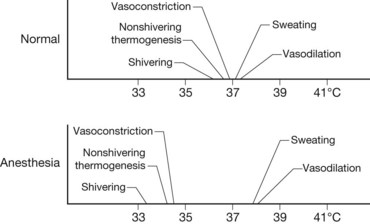
Figure 14.6 Effects of anesthesia on thermoregulatory mechanisms.
(From Sessler DI. Temperature monitoring. In: Miller R, ed. Anesthesia, 3rd edn. New York: Churchill Livingstone; 1990.)
Actions such as maintaining higher ambient air temperature, covering the extremities and head, applying warm blankets, using radiant heaters and forced air warming devices, warming fluids and blood, and warming gases are usually effective in maintaining core temperature if applied aggressively. Ideally, hypothermia should be corrected prior to transport to the operating room.131 Hypothermia revealed in the preoperative evaluation may be due to inadequate resuscitation or metabolic instability. Either situation may predispose burn patients to intolerance of anesthetic drugs or the stress of surgery.
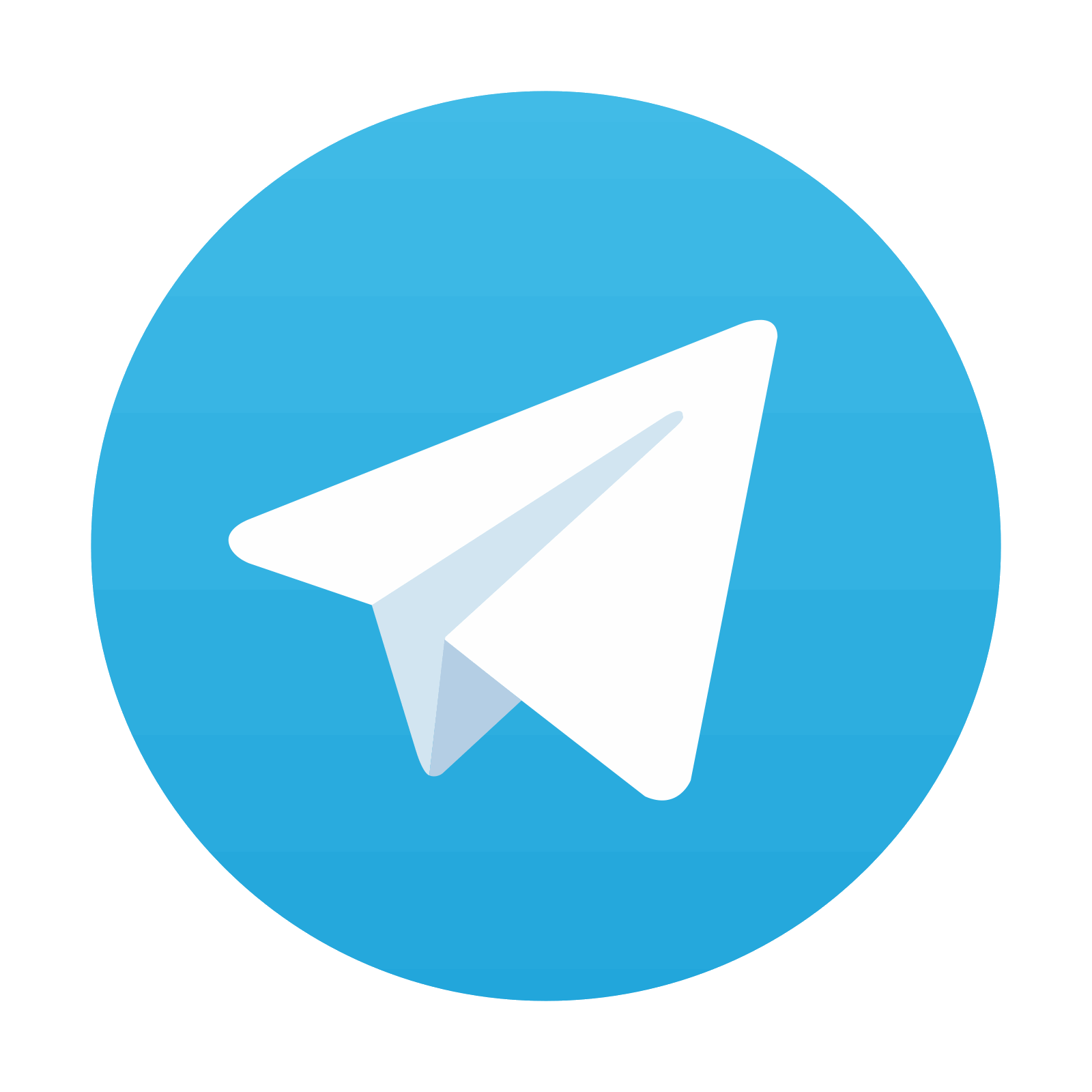
Stay updated, free articles. Join our Telegram channel
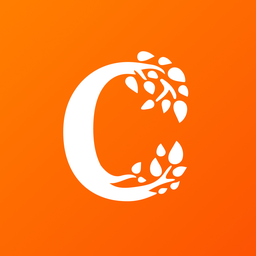
Full access? Get Clinical Tree
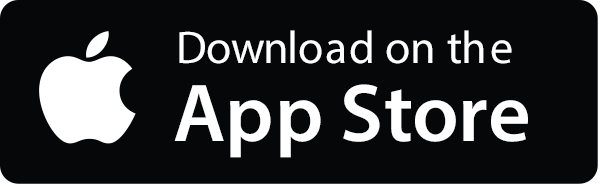
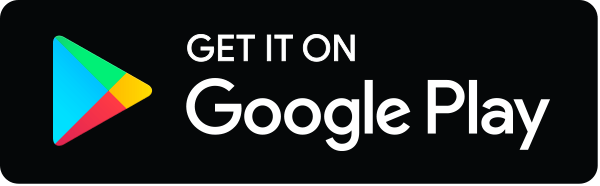