PROPERTIES OF HAIR AND THEIR RELATION TO
COSMETIC NEEDS, PERFORMANCE AND PROPERTIES
Author
Dr. Manuel Gamez-Garcia, Ph.D.,
Research Fellow,
Ashland Specialty Ingredients
ABSTRACT
In this chapter we present an unusual approach to the subject wherein we consider the system of hair structure and its relation to hair function. The approach used for this purpose consists in reviewing the most recent and advanced hair structural concepts derived from the fields of chemistry and biology, while providing a strong emphasis on the physical, social, and cosmetic aspects of hair function.
The chapter is divided into six parts:
- ● The four first sections describe the chemical and biological composition of hair, which forms the foundation for its complex physical architecture. Through these sections a detailed analysis is made of the hair internal structure, its viscoelastic and optical properties, and its response to moisture. Wherever possible an effort is made to always connect physical properties and related processes with the functional aspects of hair.
- ● In the last two sections, a review is made of the various processes taking place in the follicle to create hair. We point out that the follicle is alive, and that the native proteins in mature hair still have biological activity and a function to play. This provides access to cosmetic approaches to improve its beauty, and of course, it is a relatively new concept since the hair protein architecture has long been considered as a structure with no biological activity. There a description is made of the main biological activities occurring in the various zones and phases of the follicle to assemble cells and produce hair. As this description is made, special attention is given to the follicular activities that create, from its inception, the foundations that correlate hair architectural structure with hair function. We assert that this unique approach and a true understanding of the relationship between hair architecture and functional biology opens an entirely new field for the development of new concepts and products for the cosmetic and personal care industry catering to both men and women.
3.3.1.2 Chemical composition of hair
3.3.1.3 Main types of hair cells
3.3.1.4 Cortical cells and their role in hair properties
a. Cortical cell structure and composition
b. Viscoelasticity in hair and cortical cells
c. Shape-memory properties of hair
d. Viscoelasticity and the shape-memory properties of hair
e. Water and moisture absorption/desorption by hair
and cortical cells
3.3.1.5 Different types of cortical cells and hair shape
3.3.1.6 Cuticle cells and their role in hair properties
a. Cuticle cell structure and composition
b. Viscoelasticity in hair and cuticle cells
c. Water and moisture absorption/desorption by cuticle cells
d. Optical properties of cuticle cells
3.3.1.7 Melanin pigments in hair
a. Different zones in the follicle
It is a general principle of nature that any living organism, or biological material created by nature, is made and structured in such a way that its properties are optimized to perform a specific function. In this sense, hair is not different from any other biomaterial found in the organs of our body. Contrary to the common belief that hair is a dead material and of little or no importance, hair is an optimized assembly of proteins, lipids, and polysaccharides organized into units or cells for a specific function. All cells in our body undergo a life cycle and are created to fulfill a function. However, in this respect there is a considerable difference between hair cells and other organ cells. Cells from other parts of the body undergo a programmed form of cell death, known also as “apoptosis,” once they have fulfilled their function. After this event, the cells are discarded and eliminated from the body. In contrast, when hair cells die by “apoptosis” in the follicle, their function is not finished; rather, it has just begun. Thus, when hair cells die, they are not discarded by the body because it is then that they are needed the most for their specialized biological function of scalp/skin protection and aesthetic purposes. The short-lived period of hair cells in the follicle is just a preparatory stage for their final function.
Another common belief is that since hair cells are inanimate or lifeless, their structure has no biological activity. This statement is somewhat incorrect because the protein structure of inanimate hair cells still possesses biological activity that is crucial for our living organism. Even when hair cells are dead and inanimate, if their protein structure is damaged, as in the case of enzyme structures, their biological function is compromised. Let us remember that enzymes are lifeless protein structures whose activity plays a critical role in many of our biological processes. Enzymes are not living organisms, and they lose their biological activity when their natural conformations are lost by denaturation due to harsh stresses, either thermal, chemical, or mechanical (1).
Likewise, the proteins composing our hair cells also lose their biological function/activity when they are subjected to harsh stresses, i.e., heat, chemical treatments, mechanical fatigue, or excessive mechanical elongations. Hair treatments with hot irons and with other chemical processes such as perm waving, bleaching, and alkali straightening are known to cause denaturation and bond breakage in hair’s native proteins (2). As a consequence, many hair properties are compromised, i.e., hair loses the ability to control moisture absorption. Its mechanical/thermal/optical response is compromised, and ultimately it loses its aesthetic purpose and ability to protect the scalp. Thus, the chemical composition and protein architecture of inanimate hair cells still possess biological activity enabling hair to fulfill the biological function for which it was created.
3.3.1.2 STRUCTURE AND CHEMICAL COMPOSITION OF HAIR
Hair is composed of proteins and a very small quantity of lipids and polysaccharides. These biomaterials form the hair cells, which are spatially separated and sequentially organized in a brick-and-mortar fashion in the cylindrical hair body. Consequently, they form membranes, amorphous and crystalline protein regions that extend all along the hair fiber, giving rise to the complex architecture of hair (see Figure 1). As will be seen later, these structures are the foundation of hair’s remarkable physical properties. Table 1 displays the overall amino acid chemical composition of hair (3); the data in this table clearly show that hair is rich in half-cystine, serine, glutamic acid, and proline, i.e., the amino acids characteristic of a protein known as keratin. The next most abundant materials in hair, although at a smaller scale compared to the amount of protein, are lipids and polysaccharides, which account for 2–10% of the fiber weight. These materials, as will be discussed later, mainly appear in the cement layer gluing cortical and cuticle cells, as well as on the hair surface.
Another substance that is always present in hair is water. Incidentally, water is never considered part of the hair composition. Surprisingly, many scientists consider water to be an integral part of most biologically active proteins and, therefore, for all practical purposes water is also part of the hair composition. Native proteins contain a substantial amount of water in two forms, namely bound and free water. Free water, also referred to as “bulk water” or “loose water,” has only a minor influence in the native structure of proteins. In contrast, “bound water,” with its strong binding physical forces, attaches to specific protein groups stabilizing the protein secondary structure (4). Bound water plays an important role in the conformation of exposed side chains, and because of its role on protein stabilization many scientists consider it to be part of the protein structure. Water is thus also part of the hair keratin composition (5) because it is needed for its stability. The role of water on hair behavior will be discussed in further detail later in this chapter.
Percentages amino acids in hair:
Alanine4.6
Arginine5.8
Aspartic acid4.9
Half-cystine17.8
Glutamic acid11.4
Glycine6.4
Histidine0.9
Isoleucine2.6
Leucine5.8
Methionine0.6
Phenylalanine1.6
Proline8.4
Serine11.7
Threonine6.8
Tyrosine2.0
Valine5.8
Table 1) Amino acid composition of unaltered human hair (3)
Figure 1: Diagram of a hair fiber showing its various components (60).
3.3.1.3 MAIN TYPES OF CELLS IN HAIR
A hair fiber is mainly composed of three types of cells, namely, cortical, cuticle, and medulla cells (see Figure 2). These three types of cells are made, organized, and assembled to form hair fibers by a tiny organ called the follicle (see Figure 3). This small organ sits on the scalp, and there are as many follicles as hair fibers. While hair cells are still alive in the follicle, they acquire a protein structure, composition, and architecture that prepare them for their final function. Before the hair cells undergo apoptosis the follicle carefully feeds and instructs them so they can arrange their internal protein structures into highly specialized conformations. For instance, a large percentage of the proteins found in the cortical cells of hair are arranged into a type of peptide sequence and conformation that is unique among all proteins. This unique protein is called keratin and its helical structure is such that, later, it allows hair to attain a high degree of mechanical strength (see Figure 4).
Keratin is a protein designed by nature to provide mechanical strength wherever needed in living organisms (6). Keratin proteins are usually coiled into filaments and constitute the micro-skeleton that gives mechanical stability to many cells. Without keratin filaments, many living cells would burst or collapse under the action of fluid pressure; i.e., in the liver they act as scaffolding structures that provide mechanical strength to the hepatocytes (7). Depending on their mechanical characteristics, keratins can be divided into alpha and beta keratins, or soft and hard keratins, respectively. Alpha or soft keratins are mostly found in hair, nails, skin, other body cells, wool, and the fur of other mammals, while the hard beta keratins are typical of reptile claws, the shell of turtles, etc. (8). Alpha keratins are also classified as type I (acidic) and type II (basic) keratins, and, in hair they are always coiled in pairs (9–10). Keratins can vary in structure and molecular weight, and therefore, there is a large variety of them; however, regardless of their type, nature always uses them when there is need for mechanical strength.
Figure 2: Pictographic representation of three types of cells in hair (60).
Figure 3: Diagrammatic representation of hair follicle with its main general components and the lipid gland (62).
Figure 4: Helical structure of alpha helix in keratin.
3.3.1.4 CORTICAL CELLS AND THEIR ROLE
IN HAIR PROPERTIES
a. Cortical cell structure and composition
The cortical cells are elongated and spindle-like in shape; they constitute the major cellular component of hair fibers and are responsible for most of the mechanical properties of hair (10–11). Their irregular circular shape in a grown hair fiber has an average diameter of approximately 20 µm; their entire body length is approximately 100 µm long (see Figure 2). The cortical cells in grown hair fibers are not alive. However, before the hair fibers emerge from their follicles there is a point at which they are still alive. The main task of these cells during their life period is to synthesize keratin proteins in their bodies by assembling key amino acids into helical structures, strand them into filaments—also called intermediate filaments (IFs), and finally, bind them via disulfide bonds to an amorphous protein matrix to form macrofibrils (11–14). The amorphous protein matrix gluing the microfibrils will then be cross-linked with disulfide bonds and hardened as the cells die. The keratin filaments are crystalline in nature and are known to possess remarkable mechanical strength. To further maximize the mechanical resistance of the nascent hair fiber, the follicle facilitates the creation of hundreds of cortical cells and instructs them to interdigitate and produce an extracellular material, the cell membrane complex (CMC), which glues them all together in a cylindrical shape (Figure 5).
Figure 5: Schematic representation of cell membrane complex in hair fiber.
Because the cortical cells contain high levels of keratin, the resistance of a hair fiber to mechanical breakage is comparable to that of a copper wire of the same diameter. Yet, in spite of this remarkable mechanical strength, the hair fibers do not behave like rigid metallic wires. We all know that hair fibers on the scalp are rather soft and easy to bend and move. This motion allows them to separate and create inter-fiber pockets of air needed for sweat transfer, thermal insulation, scalp protection, and aesthetic purposes (15, 16). Unlike a bundle of copper wires, hair is known for exchanging moisture with the environment by a physical process of absorption and desorption that involves heat transfer with the environment. This process, which occurs also in wool fibers, allows them not only to act as insulating materials, but also as heat buffers that effectively regulate skin/scalp temperature (17, 18). Furthermore, from a cosmetic point of view, hair fibers do not behave like copper fibers since an assembly of hair fibers can be deformed or styled into any desired shape with the aid of water, and it can later recover its original shape.
This particular behavior of hair, which combines mechanical strength with softness and moisture absorption/desorption with heat exchange, is at the base of its biological and cosmetic function. This combination of properties results from the biological activity of hair proteins with their unique architecture within both cortical and cuticle cells. The biggest contribution to this behavior comes, however, from hundreds of keratin micro-fibrils embedded in a protein-amorphous matrix in the cortical cells. The keratin micro-fibrils have a high degree of molecular order. Being crystalline, they do not swell in water, while the amorphous phase has no order, and a part of its structure is highly swellable by water. In terms of strength, the combination of keratin micro-fibrils embedded in an amorphous phase is akin to that of an epoxy composite material reinforced with glass fibers. In this sense a single hair fiber can be considered as an amorphous protein matrix reinforced with keratin micro-fibrils.
b. Viscoelasticity in hair and cortical cells
In 1959 a mechanical model was proposed by Max Feughelman (15, 20, 21) to explain the remarkable relationship existing between elasticity, softness, strength, and moisture displayed by wool and hair. This scientist considered that the outstanding mechanical behavior of hair and wool and, in general, of most keratin fibers, results mainly from the mechanical response of keratin micro-fibrils, or intermediate filaments (Ifs), embedded in an amorphous protein matrix forming macro-fibrils in the cortical cells.
In order to explain the time-delayed elasticity associated with hair softness and its dependence on moisture, Max Feughelman introduced the concept of viscoelasticity to keratin fibers. He proposed a two-phase mechanical model that didn’t take into account the discrete nature of cortical cells, cell membrane complex (CMC), and cuticle cells. He thus generated a model of the hair fiber as if it were a continuum material—made only of keratin micro-fibrils embedded in an amorphous protein matrix (see Figure 6). In this model, now supported by a large amount of experimental evidence, the keratin micro-fibrils (C phase) are considered to act mechanically like rods in parallel with the amorphous phase (M phase M in which they are embedded) (15).
Figure 6: Representation of Max Feughelman mechanical model of keratin fibers showing keratin rods embedded in an amorphous matrix (20).
Since the introduction of the Max Feughelman model, three other models have been proposed to explain the mechanical behavior of keratin fibers. The main differences among all them seem to center on the way the amorphous phase interacts with the crystalline phase. A more detailed discussion of these differences can be found elsewhere (21). For the purpose of this chapter it suffices to emphasize, however, that all of these models recognize the existence of the amorphous and crystalline phases as two separate entities with different mechanical behavior. In all these models it is agreed that the amorphous protein phase corresponds to the zone of scrambled proteins surrounding the keratin micro-fibrils. The proteins in this zone constitute the “cement” that supports and interconnects, via disulfide bond cross-linking, hundreds of micro-fibrils to form the macro-fibrils we know as “hair.”
Within the amorphous matrix, the two-phase mechanical model recognizes the existence of two regions with different cystine content, namely, the high- and low-sulfur regions. The low-sulfur amorphous region is considered to be in close proximity to the micro-fibrils, while the high-sulfur region is further away from them. Furthermore, the amorphous phase contains hydrophilic and hydrophobic protein groups that form extended structures all along the cortical cells. Because of these two structures, the amorphous phase can display two distinct patterns of physical behavior. The first one consists of a softening effect usually observed when the amorphous phase interacts with water. The softening effect is due to the hydrophilic extended structure of the amorphous phase and is also referred to as the “gel structure” by Max Fueghelman (20). This structure absorbs large amounts of water, is susceptible to plasticization by moisture, and its viscoelastic response is moisture dependent. In contrast, the non-gel structure of the amorphous phase is hydrophobic, highly cross-linked, and softens at very high temperatures.
Phase C comprises the crystalline keratin rods forming the macro-fibrils. As a result of their crystalline character, these rods do not to absorb water, and therefore, cannot be plasticized by water. The macro-fibrils thus act like strong micro-springs that respond only elastically to mechanical stresses. Thus, macro-fibrils and amorphous phases combine their mechanical and water absorbing/desorbing properties to make hair a viscoelastic material. Because the content of crystalline keratin macro-fibril protein in hair is approximately 95%, a hair fiber has a melting temperature (Tm) of ~ 156°C. Hair has also a glass transition temperature (Tg) of ~ 144°C, and it is primarily related to the noncrystalline amorphous matrix (23). Let us not forget that the Tg in polymers or biopolymers is nothing but the temperature at which a hard amorphous polymer becomes softer (24). Therefore polymers that are very hard and brittle at room temperatures can be softened, by raising their temperature to their respective Tg. They can also be softened by adding a plasticizer. In fact, hair fibers are biopolymers, which under dry and room-temperature conditions are mechanically hard but can become softer, either, by raising their temperature above 174°C, or by adding a plasticizer. We are all familiar with the fact that water is the natural plasticizer for proteins. Thus, hair without moisture is hard and brittle, but when it absorbs moisture it becomes softer because the gel structure of the amorphous phase becomes plasticized by water. This plasticization effect by water is clearly shown in Figure 7, where it can be seen that the amount of force needed to deform a hair fiber is always lower when it has higher levels of moisture.
Figure 7: Typical stress-strain deformation curves of hair fibers obtained at three different moisture conditions (60).
The effects of water plasticization/softening in hair are more significant when hair fibers are deformed below 2% water level. The reason for this is that the main hair component that responds to low deformations is the gel structure of the amorphous phase with its large number of hydrogen bonds. At deformations (d), 2% > d < 40% the mechanical response of the fiber is not influenced by the presence of water. Rather, it is mainly controlled by the unfolding of the alpha-keratin into the beta-keratin configuration. Unfolding of the alpha helical keratin in the micro-fibrils thus allows for larger deformations in the hair fiber, thereby preventing it from immediately breaking in the yield region. The transformation of keratin from the alpha to beta configuration is a thermodynamic phase transition and can be induced in hair by many processes other than mechanical extension. When it is produced by heat or chemical damage, it is usually irreversible; however, when it is induced mechanically it is fully reversible. The beta configuration of keratin sometimes is also called the “ladder or platelet” keratin structure (22, 23). Deformations larger than 5%, giving rise to keratin unfolding, occur in hair in daily grooming practices. They occur mostly upon repetitive combing, in particular, when the hair is wet or when the hair is still wet and is being detangled.
The stress/strain curve of hair is shown in Figure 8, where it can be seen that when we deform a single hair fiber from 0 to approximately 40% in length, the hair displays three different slope regions of force versus deformation. The first line is comprised between 0 and 2 or 5% deformation depending on the relative humidity of the environment (segment A). It is known as the Hookean region and has the steepest slope. This region represents the stress/strain mechanical response of the amorphous and crystalline (alpha keratin) phases. In particular, it represents the mechanical response of protein region with hydrogen bonds. Since these levels of deformation are easily attained with everyday combing and grooming practices, we stress the amorphous and macro-fibril phases of our hair almost everyday. If we continue to deform the hair fiber between 2 and 35% (segment B), the slope stress/strain changes because it is produced by a different phenomenon, namely, the opening of keratin coils in the macro-fibrils. This section of the stress-strain curve of hair is known as the yield region. Hair deformations falling in this range are typical during excessive tangling when the hair is wet. Deformations higher than 35% cause the final change in slope before the fiber breaks; the physical phenomenon associated with these deformations seems to be a process of recrystallization of the amorphous phase (segment C) (11).
Figure 8: Stress strain curve for hair up to 30% deformation.
c. Shape-memory properties of hair
It is known from the field of polymer science that the mechanical behavior of polymers can be characterized as either being of the thermoset or thermoplastic type. Thermoset characteristics are those that do not respond to temperature changes, while thermoplastic characteristics refer to those materials that soften when heat is applied.
When polymers contain a high level of cross-linking bonds, they are usually of the thermoset type. In many cases these materials behave also as “smart” materials displaying shape-memory properties (24, 25). Since approximately 90% of the hair is composed of cortical cells filled with crystalline and amorphous proteins cross-linked by disulfide bonds, hair like any other polymeric cross-linked material behaves also as a thermoset, viscoelastic, shape-memory biopolymer. Thus, hair has a permanent shape that may be temporarily changed, but can also be reversed later under the appropriate conditions. For instance, whether the hair is limp, wavy, curly, or extremely curly, it will always remember its native shape regardless of what type of styling shape has been imposed on it. Furthermore, the fact that the hair geometry can successfully be changed after styling, and held there for a certain period of time without the hair immediately recovering its shape, is by far one of the most important cosmetically useful examples of hair viscoelasticity and memory-shape properties. If the behavior of hair were purely elastic, hair would not be able to easily deform and accommodate for deformations needed for fiber separation, air pocket formation, thermal insulation, scalp protection, and aesthetic purposes. Also, hair would not be able to transiently change its shape during styling for cosmetic purposes. Thus, hair is a viscoelastic shape-memory material, and failure to comprehend this will hamper our understanding of one of the most fundamental properties of hair’s biological and cosmetic behavior.
d. Viscoelasticity and the shape-memory properties of hair
Because of the great importance of viscoelasticity in the shape-memory properties of hair, a brief review of this concept will be helpful. We are all familiar with the fact that when a small force is applied to a pure elastic material, for instance, to a rubber band, it will deform a certain short distance. Then, when we release the force, the rubber band will recover almost instantaneously to its original shape. In this process it is obvious that the energy applied to deform the rubber band is stored as mechanical tension energy, and then returned when the force is released. In contrast, in a pure viscous material this does not happen. Rather, when we apply a similar force to a viscous material, for instance, to a string of chewing gum, a small deformation is also produced. However, contrary to the elastic material, the string of chewing gum will not recover its original shape once the force is released and it will stay deformed forever. Furthermore, the energy spent to deform a viscous material like the string of chewing gum is not returned but rather, it is dissipated as heat (26). In summary, an elastic material—when deformed—recovers its shape instantaneously when the force causing the deformation is released, while a viscous material does not.
A viscoelastic material, like hair, displays both behaviors simultaneously, namely, viscous and elastic. In fact, we all have daily encounters with materials that behave viscoelastically. For instance, when we apply a force for a short period of time, i.e., for one second, to a piece of plastic film from a sandwich bag, it will deform slightly. The shape of the film will be recovered instantaneously again when we release the force. However, if we now apply the same force, but for a longer period of time, let’s say five minutes, we will still produce the same deformation, but we will notice that when we release the force, the plastic film does not recover its original shape right away. If we wait for some time and pay careful attention to the plastic film, we will notice that it slowly crawls back and eventually recovers its original shape.
In the above example, with a one-second force application, the piece of film displayed typical elastic behavior; yet in the case of a five-minute force application the same plastic film showed initially a viscous behavior followed by a delayed, gradual, and slow elastic recovery. Thus, depending on the time period of force application, the plastic film displayed either pure elastic behavior or a combination of a viscous and elastic behavior. This is the universal characteristic of viscoelastic materials, namely, they either display elastic behavior in response to rapid deformations, or display a combination of viscous and elastic behavior in response to very slow deformations, or to deformations produced by forces applied for long time (27). Hair displays exactly the same behavior.
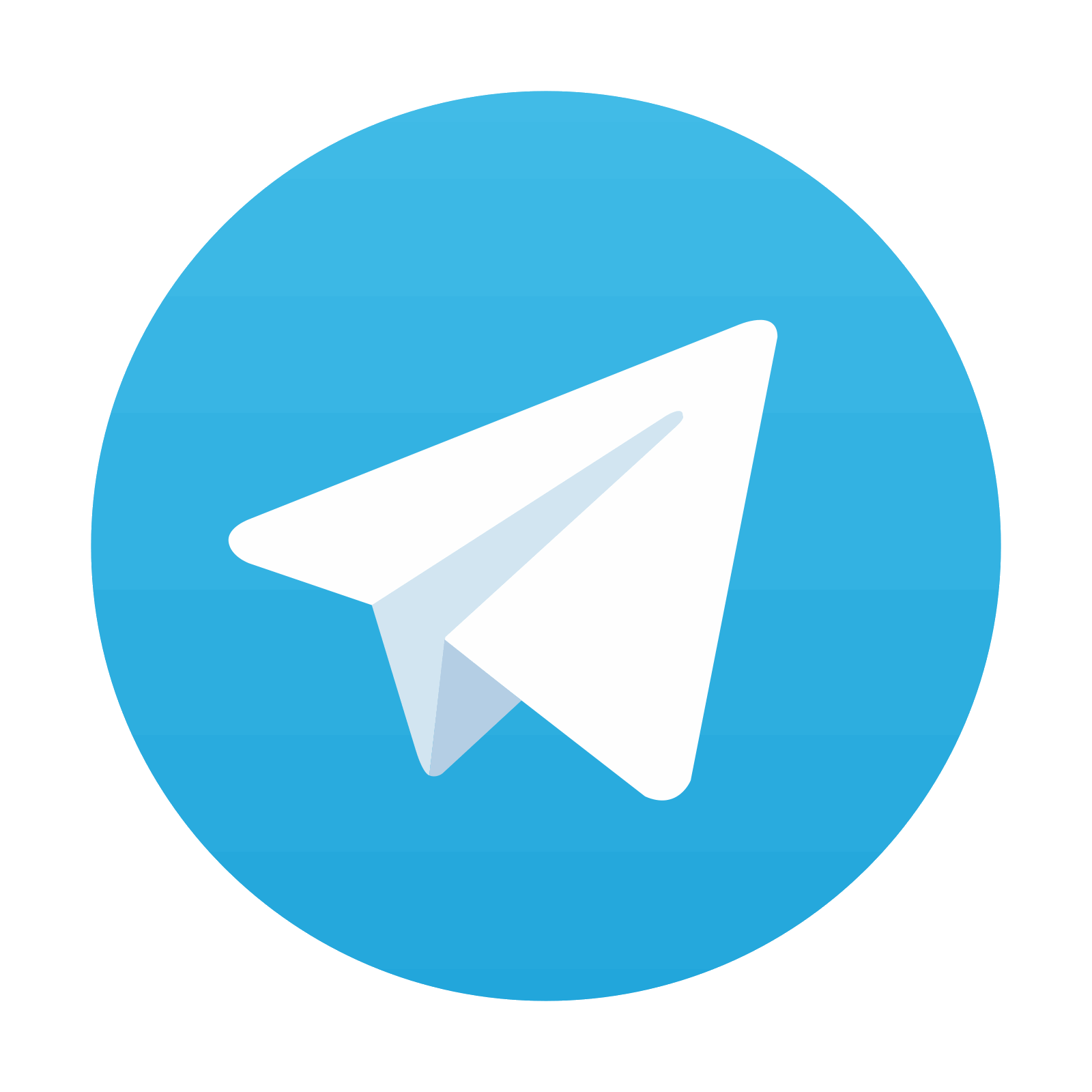
Stay updated, free articles. Join our Telegram channel
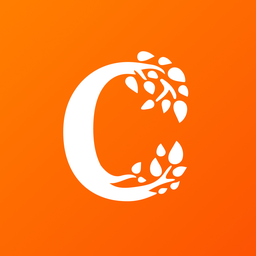
Full access? Get Clinical Tree
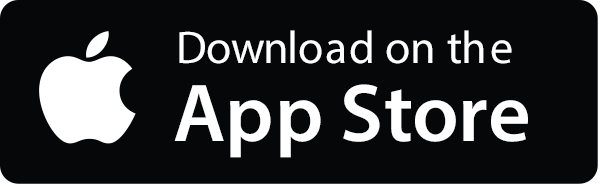
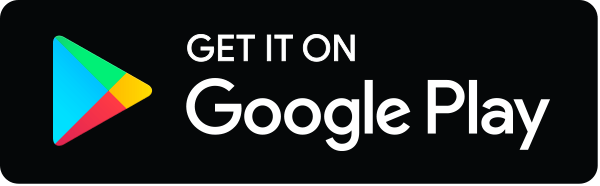