“Adipose tissue is a valuable, exploitable, appealing source of regenerative cells that can be used for a variety of clinical challenges. This article reviews the history of the development of adipose-derived cell science, particularly in the context of tissue engineering and regenerative medicine. It describes some of the advancements made in the field, as well as highlighting challenges and obstacles.”
Key points
- •
Adipose tissue is a valuable, exploitable, appealing source of regenerative cells.
- •
Adipose tissue can be used for a variety of clinical challenges.
- •
Adipose tissue has entered clinical testing throughout the world and has been shown to be safe and feasible in a variety of models.
- •
Commercial infrastructure/scalability of cell manufacturing is nearing the tipping point.
- •
Adipose-derived therapies can be developed for clinical use in a variety of ways.
- •
Adipose-derived therapies will be developed in waves of complexity.
- •
Current approaches seem to be influenced by regulatory considerations as much as by scientific considerations.
Introduction
Previously perceived as worthless and unwanted, adipose tissue has emerged over the last 15 years as a premiere source of cells for evolving tissue engineering and regenerative therapies. It was once thought to function merely as padding for internal organs, nerves, and vessels, but now adipose tissue is known to be a complex, dynamic, bioactive organ that is involved in a diverse array of physiologic and disease processes. In the 1960s, Rodbell described the dissociation and separation of adipose tissue into a single cell suspension, enabling and ushering in a new era of study of its different cellular components. This subsequently manifested as an expanded understanding of the adipocyte precursor cell (ie, the preadipocyte) and its differentiation program. In the 1980s and 1990s, the cellular constituents of adipose tissue became a central topic of study within the context of obesity, and eventually for the study and understanding of fat grafting. Ultimately, the preadipocyte became a focal point for the emerging field of tissue engineering.
As the cellular diversity of adipose tissue became more appreciated, interest in its use as a cell source increased. As early as 1986, Jarrell and colleagues published on the isolation and use of adipose-derived microvascular endothelial cells for lining synthetic vascular grafts. By the turn of the century, the idea that adipose tissue contained progenitor/stem cells with the ability to differentiate into lineages other than adipocytes emerged. In 2000, Halvorsen and colleagues described the osteogenic differentiation of adipose-derived stromal cells, and in 2001 Zuk and colleagues published findings showing that adipose tissue was a novel source of mesenchymal stem cells (MSCs) with multilineage differentiation potential. There is now a large body of literature to suggest that a wide variety of tissues may contain an MSC fraction. To this end, Crisan and colleagues, as well as several other independent groups, have proposed a unifying theory that MSCs from various organs/tissues are located in the perivascular space/niche. The notion that pericytes (also called mural cells, or Rouget cells) have multilineage potential is not new, and dates back at least to the early 1980s. Furthermore, the unique microvascular composition of adipose tissue has been appreciated for at least a quarter of a century, if not longer.
The growing global interest in the use of adipose tissue as a source of cells, matrix, and factors for regenerative and tissue engineering purposes is readily manifest in a simple PubMed literature search. As shown in Fig. 1 , the number of peer-reviewed publications involving the key words “adipose” and “stem cells” has increased greatly since 2001. A similar pattern of growth is evident in a search of the National Institutes of Health clinical trial site, www.clinicaltrials.gov , with similar terms. Coincident with these basic science and translational research advancements, a novel field or discipline emerged related to the therapeutic application of adipose-derived components (eg, cells, matrix, soluble factors). In addition, the growth and maturation of this field has created a need for an enabling clinical infrastructure that is now evident in the form of an expanding market opportunity for new and existing commercial entities. One of the major driving factors behind this commercial interest is the now full realization by the broader community of stakeholders that adipose tissue is unrivaled in its abundance, safety, appeal, and ease in terms of its harvest.

Introduction
Previously perceived as worthless and unwanted, adipose tissue has emerged over the last 15 years as a premiere source of cells for evolving tissue engineering and regenerative therapies. It was once thought to function merely as padding for internal organs, nerves, and vessels, but now adipose tissue is known to be a complex, dynamic, bioactive organ that is involved in a diverse array of physiologic and disease processes. In the 1960s, Rodbell described the dissociation and separation of adipose tissue into a single cell suspension, enabling and ushering in a new era of study of its different cellular components. This subsequently manifested as an expanded understanding of the adipocyte precursor cell (ie, the preadipocyte) and its differentiation program. In the 1980s and 1990s, the cellular constituents of adipose tissue became a central topic of study within the context of obesity, and eventually for the study and understanding of fat grafting. Ultimately, the preadipocyte became a focal point for the emerging field of tissue engineering.
As the cellular diversity of adipose tissue became more appreciated, interest in its use as a cell source increased. As early as 1986, Jarrell and colleagues published on the isolation and use of adipose-derived microvascular endothelial cells for lining synthetic vascular grafts. By the turn of the century, the idea that adipose tissue contained progenitor/stem cells with the ability to differentiate into lineages other than adipocytes emerged. In 2000, Halvorsen and colleagues described the osteogenic differentiation of adipose-derived stromal cells, and in 2001 Zuk and colleagues published findings showing that adipose tissue was a novel source of mesenchymal stem cells (MSCs) with multilineage differentiation potential. There is now a large body of literature to suggest that a wide variety of tissues may contain an MSC fraction. To this end, Crisan and colleagues, as well as several other independent groups, have proposed a unifying theory that MSCs from various organs/tissues are located in the perivascular space/niche. The notion that pericytes (also called mural cells, or Rouget cells) have multilineage potential is not new, and dates back at least to the early 1980s. Furthermore, the unique microvascular composition of adipose tissue has been appreciated for at least a quarter of a century, if not longer.
The growing global interest in the use of adipose tissue as a source of cells, matrix, and factors for regenerative and tissue engineering purposes is readily manifest in a simple PubMed literature search. As shown in Fig. 1 , the number of peer-reviewed publications involving the key words “adipose” and “stem cells” has increased greatly since 2001. A similar pattern of growth is evident in a search of the National Institutes of Health clinical trial site, www.clinicaltrials.gov , with similar terms. Coincident with these basic science and translational research advancements, a novel field or discipline emerged related to the therapeutic application of adipose-derived components (eg, cells, matrix, soluble factors). In addition, the growth and maturation of this field has created a need for an enabling clinical infrastructure that is now evident in the form of an expanding market opportunity for new and existing commercial entities. One of the major driving factors behind this commercial interest is the now full realization by the broader community of stakeholders that adipose tissue is unrivaled in its abundance, safety, appeal, and ease in terms of its harvest.
Cell identity and terminology
The stromal vascular fraction (SVF) is defined as a heterogeneous population of freshly isolated cells from adipose tissue after enzymatic dissociation ( Figs. 2 and 3 ). This cell population is composed of many different stromal cell types, such as vascular progenitors, fibroblasts, pericytes, or mesenchymal stromal cells, but excluding mature adipocytes. Within the SVF, the stromal cells of interest comprise approximately 20% of the cell fraction and have the markers CD45-, CD235a-, CD31-, CD34+; and the surface antigens CD13, CD73, CD90, CD105. In contrast, adipose stromal/stem cells (ASCs) are plastic adherent culture-expanded cells derived from SVF that possess the capability of differentiation into different mesenchymal lineages and positive expression for several immunophenotypic markers (CD90, CD105, CD73, CD44, and so forth) and are negative for CD45 and CD31 ( Fig. 3 , Table 1 ). They share these markers with other MSCs but can be differentiated from bone marrow mesenchymal stem cells (BM-MSC) based on CD36 positivity and CD 106 negativity. Furthermore, studies have suggested that ASCs have a greater propensity of differentiating into muscle tissue compared with MSCs. In contrast, MSCs have been observed to more readily differentiate into chondrogenic and osteogenic lineages.
Authors, Reference, Year | Positive Expression | Negative Expression |
---|---|---|
Gronthos et al, 2001 | CD9, CD10, CD13, CD29, CD34, CD44 CD49d, CD49e, CD54, CD55, CD59 CD105, CD146, CD166, HLA-ABC | CD11a, CD11b, CD11c, CD31, CD45 CD50, CD56, CD62e, HLA-DR |
Zuk et al, 2001 | CD13, CD29, CD44, CD49d, CD71 | CD14, CD16, CD31, CD34, CD45 |
Zuk et al, 2002 | CD90, CD105, STRO-1, SH3 | CD56, CD62e, CD104, CD106, SMA |
Katz et al, 2005 | CD29, CD49b, CD49d, CD49e, CD51 CD61, CD90, CD138, CDl40a | CD11a, CD11b, CD11c, CD18, CD41a CD49f, CD62L, CD62P, CD106 CD117, CD133, HLA-DR, ABC |
Mitchell et al, 2006 | CD13, CD29, CD34, CD44, CD49a CD63, CD73, CD90, CD146, CD166 | CD31, CD144 |
Yoshimura et al, 2006 | CD34, CD90 | CD31, CD45, CD105, CD146 |
Oedayrajsingh-Varma et al, 2007 | CD34, CD54, CD90, CD105, CD117 HLA-ABC, HLA-DR | CD31, CD45, CD106, CD146, CD166 |
Zannettino et al, 2008 | CD44, CD90, CD105, CD106, CD146 CD166, STRO-1, 3G5 | CD14, CD31, CD45 |
Traktuev et al, 2008 | CD10, CD13, CD34, CD90, CDl40a CD140b, SMA | CD31, CD45, CD144 |
Zimmerlin et al, 2010 | CD34, CD90 | CD31, CD146, SMA |
Lin et al, 2008 | CD34 | CD31, CDl40b, SMA |
Proposed phenotype of ASCs (common between 2 or more studies) | CD13, CD29, CD34, CD44, CD49d CD54, CD90, CD140a, HLA-ABC | CD11a, CD11b, CD11c, CD14, CD31 CD45, CD106, CD144 |
Controversial markers in ASCs | CD105, CDl40b, CD146, CD166, SMA HLA-DR | — |
Stromal cell markers | CD29, CD44, CD73, CD90, CD166 | — |
Hematopoietic markers | CD31, CD34, CD45, ABCG2 | — |
Pericyte markers | CD146, Stro-1, 3G5 | — |
In short, subcutaneous human adipose tissue is an accessible and abundant cell source for regenerative medicine applications. Researchers and biotech companies have explored different methods and point-of-care devices to isolate regenerative cells from human adipose lipoaspirates obtained from liposuction procedures. The body of literature has provided evidence about safety and efficacy of fresh SVF cells and cultured ASCs using in vivo animal models, as well as in different clinical studies and case reports.
Differentiation Potential
In vitro studies using human and rodent ASCs have effectively shown the trilineage differentiation potential of these cells. ASCs induced with dexamethasone undergo osteogenic differentiation leading to production of various growth factors similar to mature cells of this phenotype. Osteogenic differentiation is also seen in ASCs grown in two-dimensional and three-dimensional (3D) tissue engineered constructs using materials such as collagen, poly lactic-co-glycolic acid (PLGA), carbon nanotubes, silk sponges, or self-assembling spheroids ( Fig. 4 ). Furthermore, successful osteogenic differentiation is also seen in ASCs isolated from 24-hour-old cadaveric tissue. In vivo osteogenic differentiation of ASCs has been successfully shown in calvarial, maxillomandibular, and long bone defects. Preinduction of ASCs using dexamethasone/VD3 (1,25-dihydroxyvitamin D3) greatly improve the osteogenic capabilities of these cells compared with uninduced ASCs. The use of ASCs treated with bone morphogenetic protein 2 (BMP2) in improving bone production has shown promising results; however, there continues to be controversy about whether similar effects can be achieved by using BMP alone.
Chondrogenic differentiation is seen in ASCs grown in induction media containing members of the transforming growth factor beta family and in ASCs seeded on multiple tissue engineering constructs. In vivo chondrogenic differentiation using preinduced ASCs or ASCs transfected with TGF beta have shown production of cartilage in various in vivo models. Studies showing the successful production of articular cartilage and integration with recipient cartilage in animal models show promising clinical potential.
ASC differentiation into mature fat has been shown in in vivo models in which mature adipose tissue is generated from transplantation of green fluorescent protein (GFP)-labeled ASCs. Multiple studies also advocate the supplementation of fat grafts with ASCs to help increase take and prolong the longevity and volume retention of the grafted tissue.
Eom and colleagues showed differentiation of ASCs into skeletal muscle cells using 5-azacytidine and basic fibroblast growth factor (bFGF) induction agents. Differentiation of ASCs into myotubes has been seen when cells are grown in conjunction with myoblasts and on mechanically patterned surfaces. Successful differentiation of ASCs into smooth muscle cells and mature cardiac myocytes has been shown by the expression of mature phenotype-specific and lineage-specific markers in these cells.
In vivo differentiation in skeletal muscle tissue has been shown in studies using a subpopulation of ASCs that had been produced by rapid adherence to tissue culture polystyrene. Preinduced ASCs preferentially homed to injured muscle tissue. The cells expressed the expected muscle differentiation markers, including MyoD, myogenin, myosin, and dystrophin, along with good engraftment into target muscle tissue. Similar regenerative effects of ASCs have been seen with respect to smooth muscle cell and cardiomyocyte differentiation. Preinduced ASCs were shown to differentiate into urinary bladder smooth muscle cells and, when injected in combination with nerve growth factor, ASCs resulted in improved bladder capacity and reduced stress urinary incontinence in rats. Decreased apoptosis and increased angiogenesis were promoted in a diabetic bladder model injected with ASCs. ASCs increased left ventricular ejection fraction and improved vascularization of infarcted cardiac tissue by directly differentiating into cardiac myocytes and via expression of proangiogenic growth factors and cytokines (vascular endothelial growth factor [VEGF], bFGF, TGFb).
Ectodermal differentiation of ASCs has been shown by the creation of neurospheres and cells expressing neuronal markers (neuron-specific enolase [NSE], NeuN, nestin, microtubule-associated protein 2 [MAP2], tau, beta-tubulin III, Nkx2.2, Pax6, Olig2) and neuronlike phenotypes (delayed rectifier K currents and tetrodotoxin (TTX) sensitive sodium currents). In vivo studies have shown ASC-mediated regeneration in both central and peripheral nervous system models. ASCs reduced infarct size when injected into areas of ischemic stroke in a rodent stroke model. Improvement in extremity function and neural conduction velocities were also seen with ASC transplantation into areas of spinal contusion. In a peripheral nerve injury model, acellular conduits loaded with ASCs led to neural regeneration comparable with the gold standard of regeneration via autografts. ASCs have also been successfully induced down epidermal lineage pathways to generate epithelial-like cells and dermal stroma capable of supporting proliferation and differentiation of keratinocyte cultures.
Immunomodulation
Although differentiation-specific uses have great potential and use in tissue regeneration, ASCs also exert significant immunomodulatory effects. They have been shown to increase secretion of interleukin (IL)-6, IL-10, IL-4, and GCSF, and to stimulate proliferation of regulatory and helper T-cell phenotypes. ASC-mediated immunosuppression has led to beneficial antiinflammatory effects in hemorrhagic stroke and reactive airway disease animal models. Early results from phase III clinical trials using ASCs in the treatment of inflammatory bowel disease have shown increased healing of fistulas without any significant side effects. ASC treatments have led to improved neurologic function in patients with multiple sclerosis as well as improvements in other autoimmune disorder–related conditions such as hearing loss and rheumatoid arthritis. These successes have prompted investigation into the role ASCs could play in the prolongation of transplant-related immunosuppressive therapy and in the suppression of graft-versus-host disease.
Secretome
ASCs modify their environment via direct differentiation into target tissue cells as well as via secretion of cytokines and growth factors that exert paracrine effects. Ischemic limb model experiments comparing the effects of transplanted ASC cells with conditioned ASC media show that ASC media (containing high levels of granulocyte colony stimulating factor (GCSF), TGFB, VEGF, hepatocyte growth factor [HGF], and fibroblast growth factor [FGF]) exert similar, albeit less intense, angiogenic effects on the ischemic tissue. Similar paracrine effects of ASC-mediated proangiogenic factors have been shown in acute myocardial injury models. Immunoregulatory effects of ASC-conditioned media have been shown by the suppression of peripheral blood mononuclear cells through modulation of interferon gamma, HGF, prostaglandin E2 (PGE2), TGFB-1, indoleamine 2,3-dioxygenase (IDO), and IL-10. These effects are gaining importance in developing novel treatment modalities for Crohn disease, multiple sclerosis, and graft-versus-host disease. Conditioned media generated under proinflammatory stimuli have also shown improved wound healing rates in rodent wound healing models via the attraction of monocytes to sites of inflammation and tissue damage. Tissue regeneration via extracellular matrix (ECM) remodelling, keratinocyte migration, and increase in capillary density has been seen with the application of ASC-secreted cytokines (CTFG, SERPINE1, PAI, HGF, and FGF-1). In addition to the mechanisms summarized earlier, ASCs may also exert biological effects via direct cell-cell contacts. For example, ASCs can affect microvascular density and stability by acting as pericytes. Mendel and colleagues showed this elegantly in the context of 2 different murine models of retinopathy ( Fig. 5 ).
Scaffold-free spheroid preparation of ASCs generates media that exert more potent effects on wound healing rates than media generated by ASCs grown in monolayers. Media from 3D ASC preparations generate large amounts of ECM-related factors in addition to growth factors that promote tissue regeneration. The synergistic effects of both ECM proteins and these growth factors are considered to be one of the possible reasons for the more potent benefits observed.
In addition to relying on the innate ability of ASCs to differentiate or secrete factors in their target environment, their behavior can be engineered to secrete large amounts of specific growth factors, allowing them to behave like biopumps. The osteogenic properties of ASCs can be augmented by transducing these cells to increase production of BMP2, BMP4, BMP6, and TGF B2. Similarly, ASCs can be transduced to secrete large amounts of VEGF and brain-derived neurotrophic factor to help improve their angiogenic properties. The tumor trophic properties of these cells have been used to transmit oncolytic factors and treat gliomas. ASC cells can be converted into natural killer–like cells via the transduction of natural killer cell transcription factors in hematopoietically induced ASCs. These cells have successfully shown tumor killing properties and slowed progression of breast cancer and prostate cancer cells in a nude murine model. ASCs from both fresh and cyropreserved sources have been modified into induced pluripotent stem cells via expression of known stemness genes (Oct4, Sox2, Klf4, and c-myc). These cells form embryoid bodies in vitro that express germ cell markers including desmin, vimentin, GFAP, alpha fetoprotein, and Sox17. They assume properties of embryonic stem cells and are capable of multilineage differentiation. Dedifferentiation of mature adipocytes has been shown to produce a more potent source of ASCs. These cells retain most of the known properties of ASCs, such as their capability of multilineage differentiation, and also express stemness genes (oct4, sox2 c-myc, and Nanog).
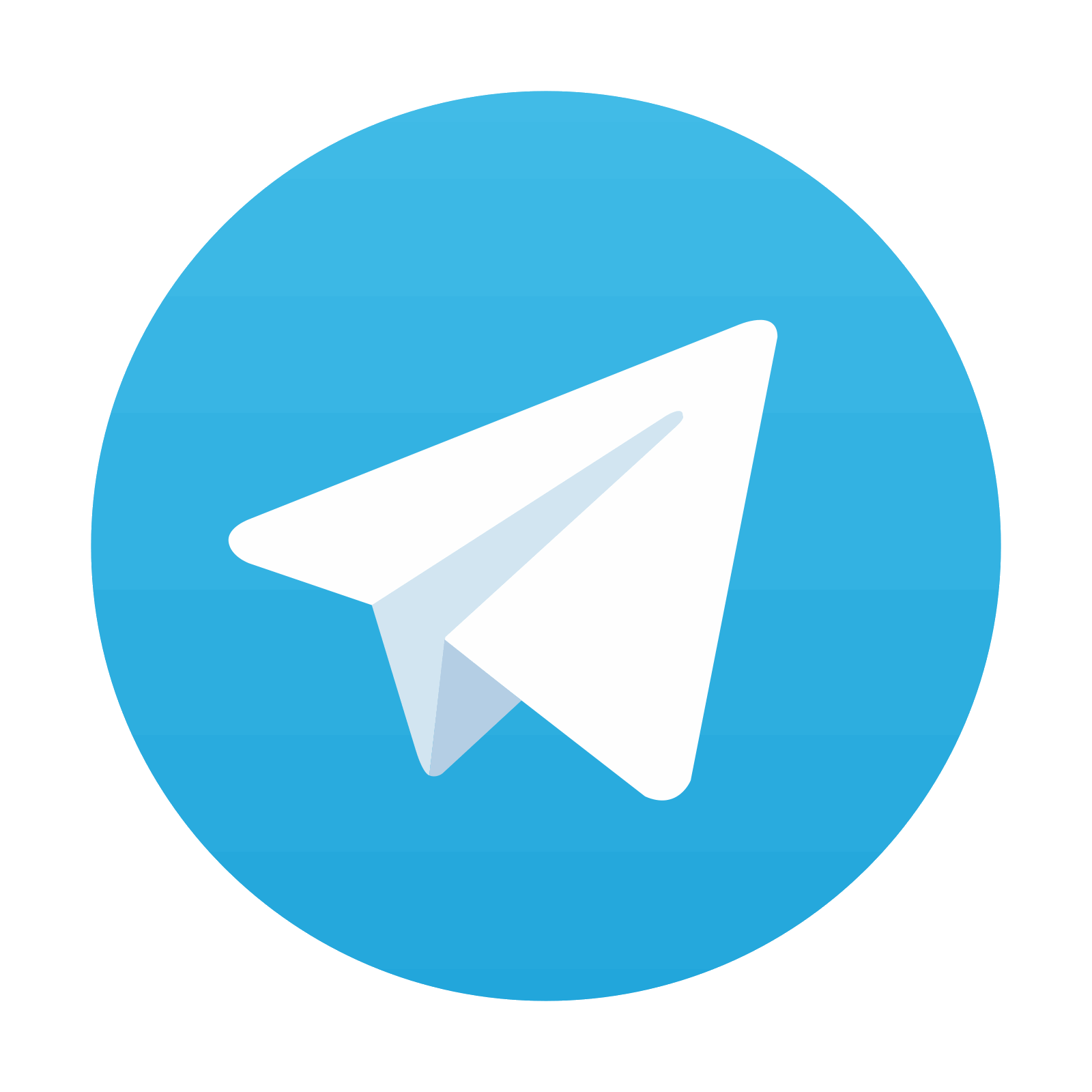
Stay updated, free articles. Join our Telegram channel
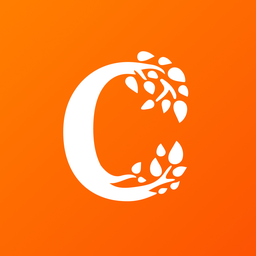
Full access? Get Clinical Tree
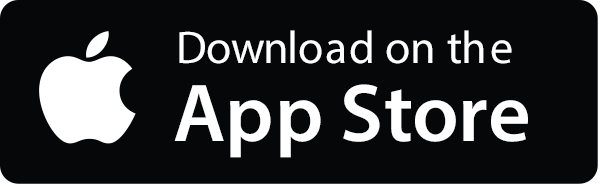
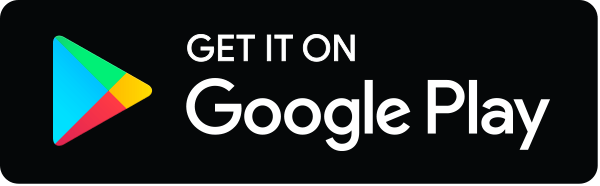